Sponsored by MerckReviewed by Emily MageeJan 23 2024
Inorganic particles often have distinct physical traits as they decrease in size toward nanometer dimensions. For instance, nanocrystalline quantum dots showcase unique electronic and optical characteristics that may find application in electro-optic devices and biomedical imaging.1
Current research, ranging from chemical sensing to magnetic recording, concentrates on leveraging the high surface-to-volume ratios of nanoparticles for constructing intricate nanomaterials.
Structures like core/shell nanoparticles and multicomponent hierarchical assemblies can display improved properties and novel functionalities due to the proximity of chemically distinct nanostructured components.
This article emphasizes the diverse range of structures and properties achievable in materials based on inorganic nanoparticles, highlighting notable questions in controlling these properties.
The discussion centers around a few illustrative examples involving insulating and metallic nanoparticles, drawing from collaborative interdisciplinary research conducted by faculty at UC Davis within the Nanomaterials in the Environment Agriculture and Technology (NEAT) organized research unit.
Understanding the origin of their unique structures and physical properties requires recognizing that nanoparticles differ from bulk materials, not just in size, but also because a significant portion of their volume is within the “hailing distance” of the surface (Figure 1).
Consequently, they can bear substantial loads of surface coatings, regions that are structurally and compositionally distinct from the bulk. These regions may include rearranged (relaxed) components of the nanoparticle structure itself, a solid shell of different compositions, or adsorbed layers of water, inorganic, or organic molecules.
The adsorbed layers can be utilized as "linkages" for constructing innovative hierarchical structures. The inherent complexities in structure, bonding, and interfacial interactions among a particle, its coating, and its neighboring environment can be harnessed to derive unique properties for various potential applications.
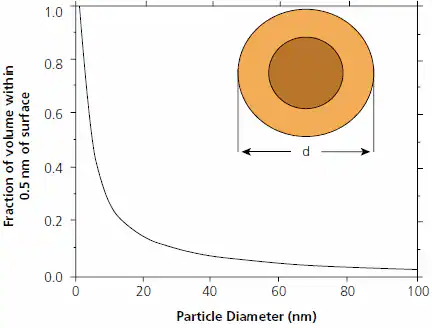
Figure 1. Fraction of volume of a particle of diameter d that lies within 0.5 nm of its surface. The lighter shell represents either that fraction or, alternately, the volume of a 0.5 nm coating on a particle of diameter d=1.5 nm. Figure modified from Ref. 15. Image Credit: Merck
Complex Multi-Phase Materials
Oxide nanoparticles, with their diverse structures and applications, are the first illustrative example to be discussed. Focusing on iron oxides and oxyhydroxides specifically, the first thing to highlight is that these materials exist in various polymorphs.2
The common anhydrous iron oxide is hematite, a-Fe2O3. Hematite is a prevalent iron ore and red paint pigment. Magnetite, Fe3O4, is a spinel and is the basis for many magnetic materials and devices, while g- Fe2O3, maghemite, adopts a defective spinel structure.
Examples of oxyhydroxides are goethite, akaganeite, lepidocrocite, ferrihydrite, and green rusts. Both oxides and oxyhydroxides often comprise nanoparticles, occurring naturally and in synthetic materials.
Iron oxide particles of different sizes are used as magnetic recording materials, ferrofluids, corrosion products, pigments, and in the transport of nutrients and contaminants (heavy metals and radionuclides) in the environment.
The magnetic properties of magnetite and maghemite are of particular interest for biological applications. However, challenges, such as corrosion sensitivity and difficulties in permanently attaching biomolecules to the nanoparticle surface, hinder these uses.
Overcoming these issues involves coating the magnetic nanoparticle with a protective shell, preventing oxidation and corrosion, and providing a platform for chemical functionalization, enhancing potential biocompatibility.
Such core/shell particles can be produced, for instance, through two successive reverse micelle reactions.3 Currently, core/shell-structured magnetic nanoparticles attract attention across various applications.4
For example, magnetic-core/Au-shell structured nanoparticles, with potential remote magnetic manipulation, may find use in biological applications such as magnetic resonance imaging (MRI), hyperthermia treatment, cell tagging and sorting, and targeted drug delivery.5-7
Several types of magnetic core/shell nanoparticles have been reported, including Fe/Au, Fe3O4/Au, and FeCo/(Au, Ag) nanoparticles.3,8-10 An example of Au-coated Fe nanoparticles is illustrated in Figure 2.3,5,11,12
Magnetic hard/soft core/shell nanoparticles, like those with FePt or CoPt in the L10 phase, are technologically significant. These nanoparticles, with high magnetocrystalline anisotropy, may be self-assembled into arrays, serving as future-generation patterned magnetic recording media with Terabit/in2 density.13
Core/shell structures combining magnetic hard phases with magnetic soft (like Fe3Pt) phases, could achieve a large energy product for permanent magnet applications.
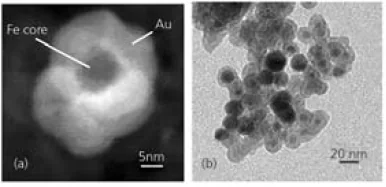
Figure 2. (a) Z-Contrast image of an Au-coated Fe nanoparticle obtained by scanning transmission electron microscopy. (b) Transmission electron microscopy image of Au-coated Fe nanoparticles. Image Credit: Merck
In this realm of research, the core/shell structure, particle size, shape, and surface properties play crucial roles.
For magnetic recording applications using each high-anisotropy magnetic nanoparticle as a single bit, minimal dispersion in size and shape distributions (<10 %) is crucial. Iron oxides, like MFe2O4 (M=Fe, Co, Mn) and Fe2O3, Fe3O4, can be prepared as monodisperse surface derivatized nanoparticles of iron oxides.14
Progress has also been made in the production of Cobalt (Co) and Iron (Fe) nanoparticles, as well as nanorods made by solution methods.
Despite these advancements and intriguing attributes, core/shell structured nanoparticles pose significant synthetic challenges, especially regarding the growth mechanisms of the core/shell structures and independent control over core/shell dimensions.
Detailed characterization of the core/shell structure, optimization of the core and shell to accomplish the required properties, and their applications in technology or biology have not yet been systematically demonstrated.
Fundamental Chemistry and Physics
Understanding the basic principles of physics and chemistry is crucial for various applications involving intricate nanostructures.
For instance, the interplay between surface and bulk contributions to a particle's energy can thermodynamically stabilize crystal structures (polymorphs) at the nanoscale, even if they are metastable in larger quantities.15-18
This phenomenon significantly influences the chemical, physical, electronic, and magnetic properties of inorganic materials.
Experimental calorimetric studies have yielded data on the surface energies and hydration energies of oxyhydroxides and iron oxides.16
Figure 3 illustrates the enthalpy of different polymorphs relative to a combination of coarse hematite and liquid water at 25 ºC. Notably, goethite becomes energetically stable compared to hematite plus water at surface areas exceeding approximately 15 m2/g (and probably is stable in free energy even at reduced surface areas due to entropy effects)
Akaganeite becomes energetically stable compared to hematite plus water at areas larger than 35 m2/g, and lepidocrocite at over 100 m2/g. Akaganeite becomes stable relative to goethite at surface areas larger than 250 m2/g.
The slope of the enthalpy-surface area line (proportional surface energy) is notably steeper for the anhydrous phase hematite compared to the hydrous phases, aligning with a broader trend also seen in the alumina system.15-19
The more metastable a polymorph, the lower its surface energy, a trend observed across iron oxides, alumina, titania, and zirconia.15 These intricate energy interactions elucidate the synthesis and coarsening behaviors of iron oxide nanoparticles in various settings, both in nature and in the laboratory.
The capacity of a particle to carry heavy metals (like uranium or lead) or organic pollutants is contingent on its crystallographic nature and surface area. The magnetic properties also hinge on phase and size.
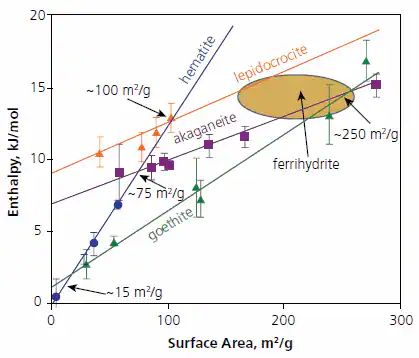
Figure 3. Calorimetrically measured enthalpies relative to coarse hematite plus liquid water ½(Fe2O3 + H2O) for oxyhydroxides and fine-grained hematite versus surface area (m2/g). The points are experimental data and the ellipse indicates the range for various ferrihydrte samples studied. Figure summarizes data taken from Refs. 16-18. Image Credit: Merck
As well as offering the framework for making "phase diagrams" to predict equilibrium nanoparticle structures as a function of their size, the measured energetics serve as benchmarks of computational results for energetics and surface structure.
Atomic-scale computer simulations taken from recent Monte-Carlo simulations of Iron-Platinum (FePt) nanoparticles) offer detailed insights into the energetic and kinetic factors governing structural transitions and stability in inorganic nanoparticles.20 Due to their unusually large magnetic anisotropy energies (MAE), and the associated very small critical sizes for superparamagnetism, FePt compounds are considered to be a promising candidate material for ultra-high-density magnetic recording devices.21
Achieving high MAE values necessitates forming FePt nanoparticles in their equilibrium tetragonal phase (L10). As synthesized, they typically adopt the metastable cubic (A1) phase, requiring a post-synthesis annealing step to induce the development of the magnetically hard tetragonal phase.
Experimental studies highlight a marked size dependence of the cubic-tetragonal, order-disorder transition temperature (TO), which is considerably reduced in nanometer-scale particles.22
Computer simulations attribute this effect to surface-induced disordering of the structure, where the particle becomes "wetted" by a disordered surface region at temperatures under the bulk TO value.
The propensity for this disordering is influenced by the surface facet’s crystalline orientation and the degree of Fe or Pt segregation onto the particle surface.
This suggests that surface coatings and control of particle morphology through the synthesis methods previously described are effective in manipulating ordering tendencies and stability of the high magnetic-anisotropy phase in small particles.
Surface Coatings and Hierarchical Assemblies
The application of surface coatings offers a route for the chemical assembly of more intricate nanostructures, such as dual-component structures with advanced uses. Examples of distinct dual-component structures with potential applications in optical waveguides, magnetic actuators, and chemical sensors are displayed in Figure 4.23
As shown in Figure 4A, magnetite nanoparticles are covalently connected to bundles of LiMo3Se3 nanowires, resulting in needle-like structures approximately 400 nm in length.
According to temperature-dependent magnetization measurements, these structures demonstrate magnetic anisotropy along their longer axis, induced by magnetic dipole interactions among the magnetite nanoparticles.
Consequently, the structures can align with weak magnetic fields, serving as nanoscale compasses24 or magnetic actuators.
Figure 4B presents a nanostructure comprising gold nanoparticles covalently linked to a colloidal plate made of HCa2Nb3O10. In this arrangement, the nanoparticle structure functions as a microscale mirror capable of reflecting light in discrete directions.25
The inset displays red, green, and yellow reflections resulting from illuminating a sample of these dispersed mirrors with two lasers. Another form of microscale waveguide is illustrated in Figure 4C.
Here, hexagonal rod-shaped zinc oxide microcrystals act as supports for covalently attached CdSe nanoparticles.26
This microstructure creates a directional emission at the rod ends when illuminated with ultraviolet light. The emission is waveguided by the ZnO microcrystal and arises from the fluorescence of CdSe quantum dots—the fluorescence wavelength is adjustable with the size of the CdSe nanoparticles.
Nanostructures in Figures 4B and 4C hold potential for spatial light modulators, light-emitting devices, and optical switches.
As a final example showcasing potential applications of hybrid multicomponent nanostructures, SiO2-Au clusters bonded electrostatically (Figure 4D) can be employed for qualitative and selective nanomolar detection of aliphatic thiols with varying hydrocarbon chain lengths (C2-C18).27
Interaction with the thiols induces changes in the binding between SiO2 and gold (Au) particles, resulting in distinct structural and color changes in the clusters. These sensors require no additional components for signal transduction and processing; thus, the submicrometer-sized clusters are genuine examples of nanoscale sensors.
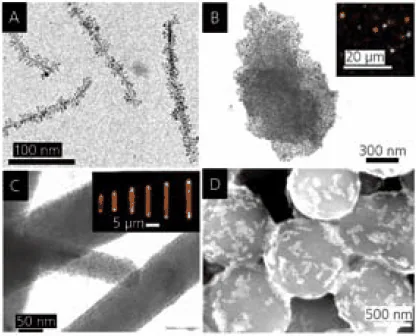
Figure 4. A. LiMo3Se3/Fe3O4 nanocompasses. B. Ca2Nb3O10/Au nanoparticle mirrors (inset: optical micrograph showing reflection). C. ZnO/CdSe light emitters (inset: optical micrograph showing directional fluorescence). D. SiO2/Au thiol sensors (after reaction with dodecanethiol). Image Credit: Merck
The provided examples underscore that the structure and properties of materials can undergo significant changes at the nanoscale—influenced by both thermodynamic factors and the kinetics of nanoparticle nucleation and growth.
The assembly of intricate hierarchical nanostructures, leading to innovative materials and devices, relies on molecular-scale interactions, particularly at the surfaces and interfaces of the nanoparticles.
A comprehensive understanding of these interactions demands a blend of experimental and theoretical techniques, while structural analysis (utilizing electron microscopy and other methods) and modeling at larger length scales are required for characterizing the formed nanostructures.
Motivated by the potential for diverse and exciting applications, this research field provides challenges in developing and integrating methodologies into a holistic picture that spans length scales from tenths to thousands of nanometers.
References and Further Reading
- Guyot-Sionnest P. 2007. Quantum Dots: An Emerging Class Of Soluble Optical Nanomaterials. Material Matters. 2(1):10.
- Cornell R, Schwertmann U. 1996. The Iron Oxides: Structure, Properties, Occurrence, Uses. VCH, Germany:
- Cho S, Kauzlarich SM, Olamit J, Liu K, Grandjean F, Rebbouh L, Long GJ. 2004. Characterization and magnetic properties of core/shell structured Fe/Au nanoparticles. Journal of Applied Physics. 95(11):6804-6806. https://doi.org/10.1063/1.1676033
- Ross C. 2001. Patterned Magnetic Recording Media. Annu. Rev. Mater. Res.. 31(1):203-235. https://doi.org/10.1146/annurev.matsci.31.1.203
- Cho S, Jarrett BR, Louie AY, Kauzlarich SM. 2006. Gold-coated iron nanoparticles: a novel magnetic resonance agent forT1andT2weighted imaging. Nanotechnology. 17(3):640-644. https://doi.org/10.1088/0957-4484/17/3/004
- Niemeyer C. 2001. Angewandte Chemie, International Edition. 404128. https://doi.org/10.1002/1521-3773(20011119)40:22<4128::AID-ANIE4128>3.0.CO;2-S
- Mornet S, Vasseur S, Grasset F, Duguet E. 2004. Magnetic nanoparticle design for medical diagnosis and therapy. J. Mater. Chem.. 14(14):2161. https://doi.org/10.1039/b402025a
- Lyon JL, Fleming DA, Stone MB, Schiffer P, Williams ME. 2004. Synthesis of Fe Oxide Core/Au Shell Nanoparticles by Iterative Hydroxylamine Seeding. Nano Lett.. 4(4):719-723. https://doi.org/10.1021/nl035253f
- Bai J, Wang J. 2005. High-magnetic-moment core-shell-type FeCo?Au?Ag nanoparticles. Appl. Phys. Lett.. 87(15):152502. https://doi.org/10.1063/1.2089171
- Cho S, Idrobo J, Olamit J, Liu K, Browning ND, Kauzlarich SM. 2005. Growth Mechanisms and Oxidation Resistance of Gold-Coated Iron Nanoparticles. Chem. Mater.. 17(12):3181-3186. https://doi.org/10.1021/cm0500713
- Liu K, Cho S, Kauzlarich SM, Idrobo JC, Davies JE, Olamit J, Browning ND, Shahin AM, Long GJ, Grandjean F. 2005. Fe-Core/Au-Shell Nanoparticles: Growth Mechanisms, Oxidation and Aging Effects. MRS Proc.. 887 https://doi.org/10.1557/proc-0887-q07-04
- Sun S, Murray C, Weller D, Folks L, Moser A. 2000. Science. 2871989.
- Sun S, Zeng H, Robinson DB, Raoux S, Rice PM, Wang SX, Li G. 2004. Monodisperse MFe2O4(M = Fe, Co, Mn) Nanoparticles. J. Am. Chem. Soc.. 126(1):273-279. https://doi.org/10.1021/ja0380852
- Navrotsky A. 2003. Energetics of nanoparticle oxides: interplay between surface energy and polymorphism?. Geochem Trans. 4(1): https://doi.org/10.1186/1467-4866-4-34
- Majzlan J, Schwertmann U, Navrotsky A. 2004. Geochemical et Cosmochimica Acta. 691039.
- Mazeina L, Navrotsky A. 2005. Surface enthalpy of goethite. Clays Clay Miner.. 53(2):113-122. https://doi.org/10.1346/ccmn.2005.0530201
- Mazeina L, Deore S, Navrotsky A. 2006. Energetics of Bulk and Nano-Akaganeite, ?-FeOOH: Enthalpy of Formation, Surface Enthalpy, and Enthalpy of Water Adsorption. Chem. Mater.. 18(7):1830-1838. https://doi.org/10.1021/cm052543j
- Majzlan J. 2000. Surface Enthalpy of Boehmite. Clays and Clay Minerals. 48(6):699-707. https://doi.org/10.1346/ccmn.2000.0480611
- Yang B, Asta M, Mryasov O, Klemmer T, Chantrell R. 2006. The nature of A1?L10 ordering transitions in alloy nanoparticles: A Monte Carlo study. Acta Materialia. 54(16):4201-4211. https://doi.org/10.1016/j.actamat.2006.05.013
- Weller D, Moser A, Folks L, Best M, Wen Lee, Toney M, Schwickert M, Thiele J, Doerner M. 2000. High K/sub u/ materials approach to 100 Gbits/in/sup 2/. IEEE Trans. Magn.. 36(1):10-15. https://doi.org/10.1109/20.824418
- Miyazaki T, Kitakami O, Okamoto S, Shimada Y, Akase Z, Murakami Y, Shindo D, Takahashi YK, Hono K. Size effect on the ordering ofL10FePt nanoparticles. Phys. Rev. B. 72(14): https://doi.org/10.1103/physrevb.72.144419
- Osterloh FE. 2006. DIRECTIONAL SUPERPARAMAGNETISM AND PHOTOLUMINESCENCE IN CLUSTERS OF MAGNETITE AND CADMIUM SELENIDE NANOPARTICLES. Comments on Inorganic Chemistry. 27(1-2):41-59. https://doi.org/10.1080/02603590500538654
- Osterloh FE, Hiramatsu H, Dumas RK, Liu K. 2005. Fe3O4-LiMo3Se3Nanoparticle Clusters as Superparamagnetic Nanocompasses. Langmuir. 21(21):9709-9713. https://doi.org/10.1021/la051498r
- Kim JY, Osterloh FE. 2006. Planar Gold Nanoparticle Clusters as Microscale Mirrors. J. Am. Chem. Soc.. 128(12):3868-3869. https://doi.org/10.1021/ja057958k
- Kim JY, Osterloh FE. 2005. ZnO?CdSe Nanoparticle Clusters as Directional Photoemitters with Tunable Wavelength. J. Am. Chem. Soc.. 127(29):10152-10153. https://doi.org/10.1021/ja052735f
- Osterloh F, Hiramatsu H, Porter R, Guo T. 2004. Alkanethiol-Induced Structural Rearrangements in Silica?Gold Core?Shell-type Nanoparticle Clusters: An Opportunity for Chemical Sensor Engineering. Langmuir. 20(13):5553-5558. https://doi.org/10.1021/la0348719
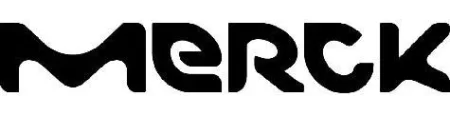
This information has been sourced, reviewed, and adapted from materials provided by Merck.
For more information on this source, please visit Merck.