Sponsored by MerckReviewed by Emily MageeJan 23 2024
Researchers in cell biology, pathology, clinical diagnostics, and drug discovery seek a deeper understanding of intracellular communication and signal networking.
Figuring out the complex interactions behind cancer, neurological diseases, and immune system disorders is especially intriguing due to their potential to unlock cures.
Studying these processes demands affordable analytical tools with high sensitivity and resolution for multiplexed analysis. Fluorescent probes, like organic fluorophores, offer many of these features and are key to pivotal discoveries.
As genomic, transcriptomic, and proteomic work uncovers more biological processes, the level of complexity surpasses the abilities of typical fluorophores. This hampers the creation of diagnostic kits and new treatments targeting crucial molecular mechanisms of diseases.
Quantum dots (QDs) developed as a result of the nanotechnology explosion in the last three decades, present a promising solution for researchers needing advanced bioassay and bioimaging technology. QDs outperform organic dyes in almost every optical aspect (Table 1).
The narrow and size-adjustable light emission makes QDs perfect for multiple imaging. The emission peaks of QDs, shaped like a Gaussian curve with an FWHM of about 20 nm, accommodate 5 to 10 colors in the visible spectrum, free of spectral overlap or resolvable by spectral imaging.1
QDs' narrow emission is supported by efficient light absorption across a wide spectral range (hundreds of nanometers), allowing simultaneous excitation of various colors by a single light source.
This not only cuts imaging instrument costs (like filter design) and simplifies data analysis (such as fluorescence intensity compensation) but also enhances imaging sensitivity, as it moves far from the autofluorescence of biological samples.
QD fluorescence can stand out from background autofluorescence using a gating approach capitalizing that exploits the longer excited-state lifetime of QDs, which is around ten times longer than organic dyes.
In theory, the extended lifetime might lower the photon production rate (and therefore the brightness of the probe). However, the majority of biological imaging applications operate under absorption-limited conditions instead of lifetime-limited conditions, which need a very intense excitation photon flux.
This makes QDs, with considerably higher molar extinction coefficients, brighter probes compared to organic dyes. When comparing organic dyes and QDs with similar quantum yields, individual QDs are usually 1–2 orders of magnitude brighter than dye molecules.
QDs also show much greater resistance to photobleaching, making them ideal for extended imaging and tracking applications.
Table 1. Comparison of organic dye and QD optical properties significant for bioapplications. Source: Merck
Optical
property |
Conventional organic dyes |
QDs |
Absorption spectrum |
Narrow in general |
Broad and gradually increasing towards shorter wavelength |
Emission spectrum |
Broad with long-wavelength tails |
Narrow, symmetrical, Gaussian distributed |
Molar extinction
coefficient |
104–105 M-1cm-1 |
105–106 M-1cm-1 at the first exciton peak, increasing towards shorter
wavelength |
Quantum yield |
High-quality dyes and QDs share similar quantum yields |
Fluorescence excited
state lifetime |
Nanoseconds |
10 nanoseconds |
Photostability |
Poor, rapid photobleaching |
Highly stable |
Stokes shift |
Small in general, often <50 nm |
Flexible depending on excitation wavelength, can be as large as 100s nm |
Size |
Small, ~1 nm |
Large, core particle often 2–10 nm in diameter; polymer coating adds
~3 nm for the hydrodynamic size |
QD Developments
Despite the impressive optical properties, it took a considerable amount of time to refine the synthesis and engineering of QDs to the point where they become practical for use in bioassays and biological imaging.
The journey from the initial discovery and establishment of quantum mechanical models for QDs in the early 1980s,2-4 to the availability of high-quality commercially viable QDs, spanned nearly two decades of development.5
This lengthy process can be roughly divided into three significant milestones: QD core synthesis, QD shell synthesis, and QD surface functionalization.
In 1993, Bawendi and colleagues6 made a breakthrough by introducing a new method to produce monodisperse QD core particles. Departing from the then-common aqueous solution-based reactions, this approach utilized high temperatures, organometallic reactants, and organic solvents.
These conditions facilitated swift crystal nucleation and allowed precise control over the competing processes of Ostwald ripening and molecular addition during nanocrystal growth.7
Consequently, this method not only yielded highly crystalline, uniform II-VI QDs of precise sizes, but also laid the groundwork for synthesizing other uniform nanoparticles, such as III-V semiconductor, magnetic, metallic, and perovskite nanoparticles.8-12
However, QDs produced using Bawendi’s method often had quantum yields below 10 %, greatly limiting the manufacture of bright imaging probes. The small particle size meant a significant portion of the atoms resided on the QD surface.
These surface atoms caused crystal defects that trapped charge carriers, hindering exciton recombination and fluorescence emission.
An ingenious solution, first introduced by Hines and Guyot-Sionnest in 1996,13 involved growing a thin shell atop the core nanoparticles. This shell consisted of another semiconductor with a similar crystal lattice but a higher energy bandgap.
It effectively passivated the core particle surface and confined excitons to the core, encouraging recombination and fluorescence emission. Initially, the core-shell QDs achieved quantum yields of 30-50 %, which was later increased to 80-90 % by implementing more intricate shells.14
The third crucial milestone needed to make QDs practical for biomedical applications involved surface functionalization. High-quality QDs synthesized in organic solvents have a layer of hydrophobic surface ligands that are not compatible with biological systems.
Preliminary attempts to make QDs water-soluble relied on ligand exchange and silica coating. However, both methods displaced the crucial hydrophobic surface ligands15,16 that contributed to QDs' optical properties. Moreover, the water-soluble QDs produced through these methods did not form stable colloids.
While freshly synthesized QDs worked for lab research and proof-of-concept studies, they were not suitable for commercial applications.
Several laboratories explored using amphiphilic polymers to render QDs water-soluble while keeping the hydrophobic ligands on the surface.17-20 These polymers functioned like detergent molecules wrapping around small oil droplets to solubilize hydrophobic QDs.
For instance, Bruchez and colleagues at Quantum Dot Corporation engineered a polymer featuring multiple repeats of hydrocarbon chains and polar head groups, such as carboxylic acids.20
These hydrocarbon chains integrated into the hydrophobic surface ligands on the QD surface, while the polar head groups rendered the QDs water-soluble (Figure 1). This successful approach soon became the foundational structure for most commercially available QD bioconjugates.
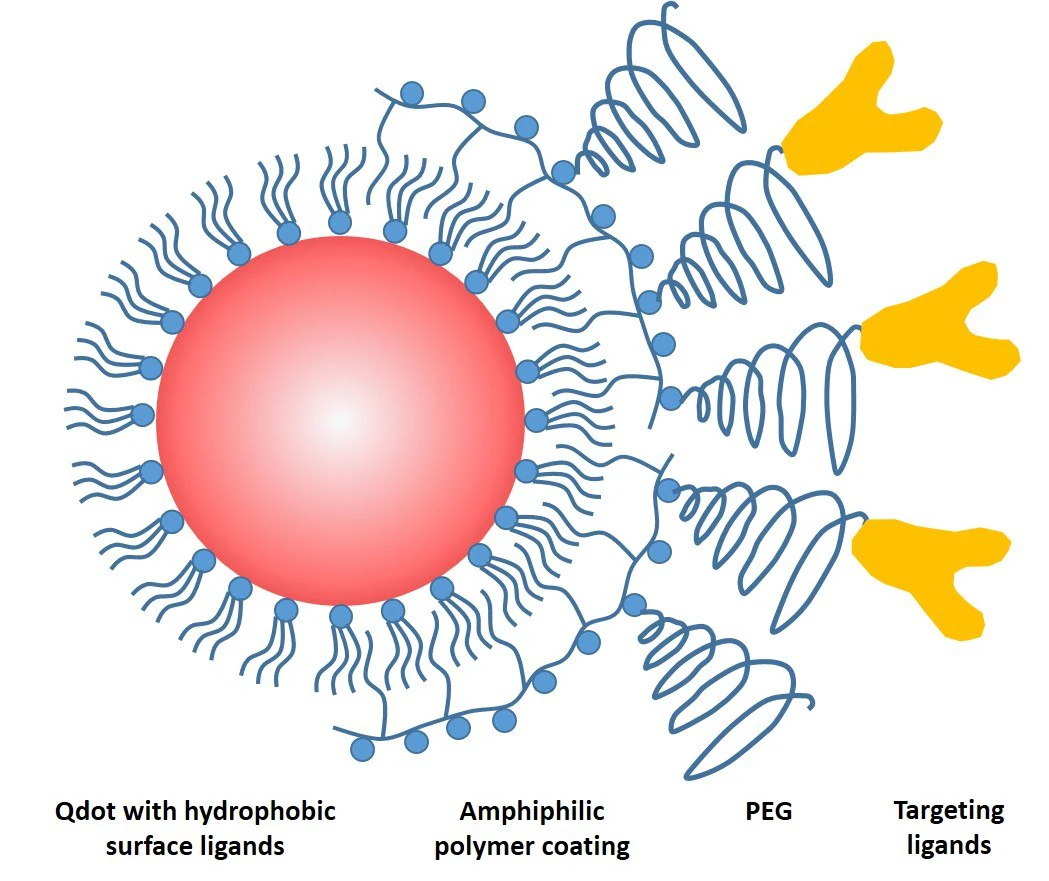
Figure 1. Schematic drawing of a common QD probe featuring a core particle capped by a layer of hydrophobic ligands, an amphiphilic encapsulating polymer layer for solubility, a polyethylene glycol (PEG) layer for non-specific binding reduction, and a targeting ligand for biomolecular recognition. Image Credit: Merck
QD Bio Applications
The applications of QDs in biology can be broadly divided into three groups: sensing and detection, in vitro labeling and imaging, and in vivo imaging. In sensing and detection, an optical readout is utilized in both homogeneous assays (detection in solutions) and heterogeneous assays (on solid supports).
The advantage of using QDs lies in their capacity to enhance detection sensitivity due to the distinctive optical features like brightness and a large Stokes shift. One of the first QD biosensors based on fluorescence resonance energy transfer (FRET) was developed by Medintz, Mattoussi, and Mauro.21
In this setup, recombinant maltose-binding protein (MBP) featuring a C-terminal histag self-assembled onto the QD surface through chelation.
A conjugate of β-cyclodextrin and a fluorescence quencher, bound within the saccharide-binding site of MBP, initially quenches the fluorescence of the QD. When present, maltose can displace the <br> β-cyclodextrin-quencher conjugate, thus restoring the fluorescence of the QD for detection.
However, using QDs in FRET assays has drawbacks. This is due to their physical size and the materials used for surface coating—particularly with highly stable amphiphilic polymer-coated QDs. These physical barriers increase the distance between energy donors and acceptors, thereby reducing the efficiency of FRET.
The diminished efficiency can be addressed by leveraging the tunable emission and ample surface area of QDs. This allows for the optimization of spectral overlaps between donors and acceptors and the immobilization of numerous energy acceptors per QD, respectively.22
Moreover, pairing QD-based FRET probes with microfluidic devices can facilitate separation-free, single molecule-level (less than 50 copies) identification of target DNA sequences. This is achievable by confining multiple oligonucleotide probes on the QD surface, capturing and concentrating numerous target molecules on the same QD.23
The second widely adopted use of QDs is in fluorescent labeling and imaging. QDs can be linked to targeting ligands such as small molecules, antibodies, peptides, and oligonucleotides. These conjugates are then employed to label specific target molecules present in paper strips, membranes, biochips, gels, or cells.
The process achieves molecular recognition through specific interactions between the target molecule and its ligand (such as antigen and antibody, or complementary DNA strands). The fluorescence helps reveal both the location and abundance of the target.
For instance, immunohistochemistry (IHC) has been a stalwart in biological research and clinical diagnosis for over fifty years. However, widely available, conventional, organic, dye-labeled antibodies (or antibody labeling kits) suffer from several limitations.
Several limitations affect the use of conventional organic dyes in labeling. Firstly, the spectral overlap restricts the use of many colors in parallel—only two to three colors can be used simultaneously.
Secondly, accurately quantifying fluorescence signals is challenging due to the rapid photobleaching of dye molecules.
Thirdly, biological samples like cells and tissues often exhibit high background signals (autofluorescence), which can interfere with or obscure specific spatial labeling.
QDs conjugated to primary antibodies offer solutions to these challenges. Individual QD-antibody conjugates are small enough to penetrate properly fixed cells. Indeed, numerous research groups have routinely demonstrated multicolor imaging of cell-surface, cytoplasmic, and nuclear antigens using QD-based approaches.1,20,24-26
Despite the advantages, QDs are not the primary choice for fluorescence labeling due to two reasons. Firstly, while QDs enhance multiplexing capabilities from 2–3 colors to 5–10, this range falls short of the comprehensive molecular profiling needed.
Secondly, the optimization and development of QD-antibody conjugation protocols,27 while advanced, require substantial labor, leading to expensive production for creating a wide array of conjugates.
In response to these challenges, Merck Millipore Sigma developed a multicolor, multicycle, molecular profiling (M3P) technology that combats these issues.28,29
To enhance multiplexing, 5–10 color QD-antibody conjugates are combined into a single cocktail. This mixture is then incubated with cells or tissue sections to showcase parallel multiplexed staining.
After the fluorescence microscopy, the stains were removed, and the sample was regenerated for another round of multicolor staining.
Merck Millipore Sigma developed protocols to completely de-stain cells without any signal carryover, preserving cell morphology and biomarker antigenicity. This allows for the subsequent full cycle of IHC staining to identify a different subset of biomarkers.
Each staining cycle enables the analysis of 10 biomarkers using 10 spectrally distinct QDs. Conducting IHC staining for 10 consecutive cycles generates 10 subsets of data for the specimen, resulting in an overall molecular profile consisting of 100 distinct biomarkers.
In each IHC staining cycle, 10 biomarkers undergo analysis using 10 different spectrally distinct QDs. Conducting 10 consecutive cycles of IHC staining yields 10 subsets of data for the sample, producing an extensive molecular profile of 100 distinct biomarkers.
In efforts to reduce the labor involved in creating custom QD-antibody bioconjugates, the team replaced the low-yield covalent QD-antibody conjugation with a non-covalent self-assembly protocol. This method joins a universal QD-protein A platform with a variety of intact primary antibodies.
This method requires no chemical reactions or purifications for end users and allows for the swift and straightforward preparation of custom QD-antibody panels, even at an extremely small scale.
The stable non-covalent interaction between the adaptor protein and primary antibodies remains intact during staining, preventing any cross-reactivity.
Consequently, the M3P technology facilitates extensive molecular characterization of cells within the native microenvironment in just a few cycles, making QDs highly practical for highly multiplexed IHC.
Moving to the third category of QD bioapplications, in vivo imaging, Dubertret et al.17 documented the initial use of QDs in a living organism—an embryo of a frog. The chemical and photostability of QDs enabled cell lineage tracking and comparative embryology studies for up to four days without causing any abnormalities in embryo development.
For in vivo targeting, Akerman et al.30 illustrated that QDs guided by peptides accumulated at tumor blood vessels utilizing ex vivo histology sections. Gao and colleagues were the first to exhibit non-invasive tumor imaging in mice using QD-antibody conjugates.18
To distinguish QD fluorescence from background signals, hyperspectral imaging was employed, particularly because mouse skin autofluorescence persisted, even when red QDs (with relatively long wavelengths and large Stokes shifts) were utilized to create the targeted imaging probe.
In efforts to enhance light penetration depth and minimize autofluorescence, Kim et al.31 developed a distinct type of QD where both the core valence and conduction band edges were adjusted to be either lower or higher than those of the shell.
This adjustment effectively red-shifted QD emission toward the near-infrared (NIR) region, albeit at the expense of exciton recombination rates.
NIR QDs injected intradermally into mice and pigs swiftly drained to nearby lymph nodes, enabling image-guided surgery.
Perspectives
The spectrum of colors made possible by QD technology and the underlying science behind it are truly captivating and beautiful.
Ever since the initial application of QDs in cell staining, they held the potential for a significant advancement in bioassay and bioimaging. However, fulfilling that potential has faced obstacles, causing delays in realizing the promise.
Consequently, QDs have encountered challenges in becoming a reliable tool for substantial biological discoveries over the last two decades. Most QD bioapplications so far have revolved around technological advancements or experimental use in model systems rather than widespread practical implementation.
As most technical challenges have been addressed, the focus in the coming years will be on identifying pivotal applications for QDs, particularly those biological problems that conventional dyes fail to adequately address.
Multicolor, quantitative biomarker imaging, including techniques like immunofluorescence and fluorescence in situ hybridization in cells and tissues, remains an incredibly exciting area for research and development.
In the realm of immunotherapy research, there is growing anticipation for highly multiplexed molecular profiling of the cell genome, transcriptome, and proteome within the natural microenvironments. This exploration is poised to unveil new insights into the intricate and dynamic human immune system.
To comprehend the interactions at molecular, cellular, and organismal levels, high-throughput and high-content molecular analysis toolkits are needed. QDs could support the development of immunotherapies that could potentially revolutionize the lives of individuals affected by various immune diseases.
In terms of in vivo applications, QD probes are anticipated to have an immediate influence on small animal imaging. For instance, in preclinical drug discovery studies, QDs present notable advantages over existing imaging methods like magnetic resonance imaging and positron emission tomography—QDs achieve high sensitivity, resolution, and cost-effectiveness concurrently.
QDs serve as an exemplary material for nanoparticle engineering. Much can be learned from QDs, including particle size, shape, charge, surface coating, and targeting ligands. This can then be applied to inform the design of other types of nanomaterials.
While the potential for in vivo use in human diagnostics and theranostics exists, its realization remains uncertain. Any application of QDs in humans must demonstrate benefits that significantly outweigh associated risks, which is yet to be substantiated.
A fundamental limitation of optical imaging lies in the shallow penetration of light through tissues. Concerns regarding QD also toxicity persist. Advanced endoscopy technologies might offer a pathway to overcome the challenge of light penetration.
To conclude, recent strides in both chemistry and colloidal science have rendered QDs a robust and accessible imaging tool. Following their commercial triumph in display technologies, widespread adoption of QDs in life sciences seems imminent.
The distinctive optical properties of QDs complement those of conventional organic dyes and fluorescent proteins, opening doors to discoveries in many unexplored or underexplored biological areas. The future looks promising for QDs.
References and Further Reading
- True LD, Gao X. 2007. Quantum Dots for Molecular Pathology. The Journal of Molecular Diagnostics. 9(1):7-11. https://doi.org/10.2353/jmoldx.2007.060186
- Brus LE. 1984. Electron?electron and electron?hole interactions in small semiconductor crystallites: The size dependence of the lowest excited electronic state. The Journal of Chemical Physics. 80(9):4403-4409. https://doi.org/10.1063/1.447218
- Rossetti R, Nakahara S, Brus LE. 1983. Quantum size effects in the redox potentials, resonance Raman spectra, and electronic spectra of CdS crystallites in aqueous solution. The Journal of Chemical Physics. 79(2):1086-1088. https://doi.org/10.1063/1.445834
- Efros A, Efros A. 1982. Semiconductors. 16(7):772–775.
- Jovin TM. 2003. Quantum dots finally come of age. Nat Biotechnol. 21(1):32-33. https://doi.org/10.1038/nbt0103-32
- Murray CB, Norris DJ, Bawendi MG. 1993. Synthesis and characterization of nearly monodisperse CdE (E = sulfur, selenium, tellurium) semiconductor nanocrystallites. J. Am. Chem. Soc.. 115(19):8706-8715. https://doi.org/10.1021/ja00072a025
- Peng X, Wickham J, Alivisatos AP. 1998. Kinetics of II-VI and III-V Colloidal Semiconductor Nanocrystal Growth: ?Focusing? of Size Distributions. J. Am. Chem. Soc.. 120(21):5343-5344. https://doi.org/10.1021/ja9805425
- Protesescu L, Yakunin S, Bodnarchuk MI, Krieg F, Caputo R, Hendon CH, Yang RX, Walsh A, Kovalenko MV. 2015. Nanocrystals of Cesium Lead Halide Perovskites (CsPbX3, X = Cl, Br, and I): Novel Optoelectronic Materials Showing Bright Emission with Wide Color Gamut. Nano Lett.. 15(6):3692-3696. https://doi.org/10.1021/nl5048779
- Battaglia D, Peng X. 2002. Formation of High Quality InP and InAs Nanocrystals in a Noncoordinating Solvent. Nano Lett.. 2(9):1027-1030. https://doi.org/10.1021/nl025687v
- Park J, An K, Hwang Y, Park J, Noh H, Kim J, Park J, Hwang N, Hyeon T. 2004. Ultra-large-scale syntheses of monodisperse nanocrystals. Nature Mater. 3(12):891-895. https://doi.org/10.1038/nmat1251
- Park J, Lee E, Hwang N, Kang M, Kim SC, Hwang Y, Park J, Noh H, Kim J, Park J, et al. 2005. One-Nanometer-Scale Size-Controlled Synthesis of Monodisperse Magnetic Iron Oxide Nanoparticles. Angew. Chem. Int. Ed.. 44(19):2872-2877. https://doi.org/10.1002/anie.200461665
- Puntes VF. 2001. Colloidal Nanocrystal Shape and Size Control: The Case of Cobalt. 291(5511):2115-2117. https://doi.org/10.1126/science.1057553
- Hines MA, Guyot-Sionnest P. 1996. Synthesis and Characterization of Strongly Luminescing ZnS-Capped CdSe Nanocrystals. J. Phys. Chem.. 100(2):468-471. https://doi.org/10.1021/jp9530562
- Xie R, Kolb U, Li J, Basché T, Mews A. 2005. Synthesis and Characterization of Highly Luminescent CdSe?Core CdS/Zn0.5Cd0.5S/ZnS Multishell Nanocrystals. J. Am. Chem. Soc.. 127(20):7480-7488. https://doi.org/10.1021/ja042939g
- Bruchez Jr. M. 1998. Semiconductor Nanocrystals as Fluorescent Biological Labels. 281(5385):2013-2016. https://doi.org/10.1126/science.281.5385.2013
- Chan WC. 1998. Quantum Dot Bioconjugates for Ultrasensitive Nonisotopic Detection. 281(5385):2016-2018. https://doi.org/10.1126/science.281.5385.2016
- Dubertret B. 2002. In Vivo Imaging of Quantum Dots Encapsulated in Phospholipid Micelles. 298(5599):1759-1762. https://doi.org/10.1126/science.1077194
- Gao X, Cui Y, Levenson RM, Chung LWK, Nie S. 2004. In vivo cancer targeting and imaging with semiconductor quantum dots. Nat Biotechnol. 22(8):969-976. https://doi.org/10.1038/nbt994
- Pellegrino T, Manna L, Kudera S, Liedl T, Koktysh D, Rogach AL, Keller S, Rädler J, Natile G, Parak WJ. 2004. Hydrophobic Nanocrystals Coated with an Amphiphilic Polymer Shell: A General Route to Water Soluble Nanocrystals. Nano Lett.. 4(4):703-707. https://doi.org/10.1021/nl035172j
- Cressier P. 2002. Prendre les eaux en al-Andalus. Pratique et fréquentation de la Hamma. medi. 21(43):41-54. https://doi.org/10.3406/medi.2002.1555
- Medintz IL, Clapp AR, Mattoussi H, Goldman ER, Fisher B, Mauro JM. 2003. Self-assembled nanoscale biosensors based on quantum dot FRET donors. Nature Mater. 2(9):630-638. https://doi.org/10.1038/nmat961
- Medintz IL, Trammell SA, Mattoussi H, Mauro JM. 2004. Reversible Modulation of Quantum Dot Photoluminescence Using a Protein- Bound Photochromic Fluorescence Resonance Energy Transfer Acceptor. J. Am. Chem. Soc.. 126(1):30-31. https://doi.org/10.1021/ja037970h
- Zhang C, Yeh H, Kuroki MT, Wang T. 2005. Single-quantum-dot-based DNA nanosensor. Nature Mater. 4(11):826-831. https://doi.org/10.1038/nmat1508
- Ghazani AA, Lee JA, Klostranec J, Xiang Q, Dacosta RS, Wilson BC, Tsao MS, Chan WCW. 2006. High Throughput Quantification of Protein Expression of Cancer Antigens in Tissue Microarray Using Quantum Dot Nanocrystals. Nano Lett.. 6(12):2881-2886. https://doi.org/10.1021/nl062111n
- Giepmans BNG, Deerinck TJ, Smarr BL, Jones YZ, Ellisman MH. 2005. Correlated light and electron microscopic imaging of multiple endogenous proteins using Quantum dots. Nat Methods. 2(10):743-749. https://doi.org/10.1038/nmeth791
- Yezhelyev M, Al-Hajj A, Morris C, Marcus A, Liu T, Lewis M, Cohen C, Zrazhevskiy P, Simons J, Rogatko A, et al. In Situ Molecular Profiling of Breast Cancer Biomarkers with Multicolor Quantum Dots. Adv. Mater.. 19(20):3146-3151. https://doi.org/10.1002/adma.200701983
- Zrazhevskiy P, Dave SR, Gao X. 2014. Addressing Key Technical Aspects of Quantum Dot Probe Preparation for Bioassays. Part. Part. Syst. Charact.. 31(12):1291-1299. https://doi.org/10.1002/ppsc.201400184
- Zrazhevskiy P, Gao X. 2013. Quantum dot imaging platform for single-cell molecular profiling. Nat Commun. 4(1): https://doi.org/10.1038/ncomms2635
- Zrazhevskiy P, True LD, Gao X. 2013. Multicolor multicycle molecular profiling with quantum dots for single-cell analysis. Nat Protoc. 8(10):1852-1869. https://doi.org/10.1038/nprot.2013.112
- Akerman ME, Chan WCW, Laakkonen P, Bhatia SN, Ruoslahti E. 2002. Nanocrystal targeting in vivo. Proceedings of the National Academy of Sciences. 99(20):12617-12621. https://doi.org/10.1073/pnas.152463399
- Kim S, Lim YT, Soltesz EG, De Grand AM, Lee J, Nakayama A, Parker JA, Mihaljevic T, Laurence RG, Dor DM, et al. 2004. Near-infrared fluorescent type II quantum dots for sentinel lymph node mapping. Nat Biotechnol. 22(1):93-97. https://doi.org/10.1038/nbt920
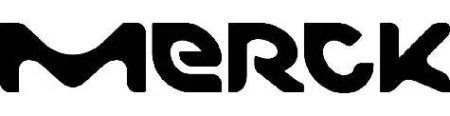
This information has been sourced, reviewed, and adapted from materials provided by Merck.
For more information on this source, please visit Merck.