Sponsored by MerckReviewed by Emily MageeJan 23 2024
Stem cell therapies show promise in tissue engineering, regenerative medicine, and their homing effect. They offer hope for treating numerous incurable diseases like Parkinson’s disease, liver failure, ischemic heart disease, and cancer.
However, understanding the cell distribution, migration behaviors, and functionality post-engraftment remains challenging due to the absence of effective in vivo tracing methods. This lack impedes the clinical progress of stem cell therapies.
To overcome this hurdle, it is crucial to develop advanced imaging strategies that can label and track transplanted stem cells without disrupting their normal functions. These strategies are necessary to uncover therapeutic mechanisms and assess safety before clinical trials.
Imaging techniques suited for stem cells should prioritize non-toxicity, high resolution, longevity, and the dynamic provision of cell fate information. Over recent years, several bioimaging approaches have emerged.
One particularly promising tactic involves leveraging well-designed nanoparticles as contrast agents to monitor stem cells. These nanoparticles boast unique physicochemical properties and offer versatility and customizability.
However, existing imaging methods still fall short of meeting all the necessary in vivo stem cell tracking demands.
This article provides a concise examination of the four commonly utilized nanoparticle-based imaging techniques for the tracking of stem cells: magnetic resonance imaging (MRI), fluorescence imaging, ultrasound imaging (USI), and photoacoustic imaging (PAI) (Figure 1).
Emphasis is placed on the design of contrast nanoagents, the corresponding imaging mechanisms, and the specific challenges these nanoagents have addressed.
The objective is to illuminate potential pathways for future advancements in contrast agent development, aiming to create more sophisticated solutions for the in vivo tracking of transplanted stem cells.
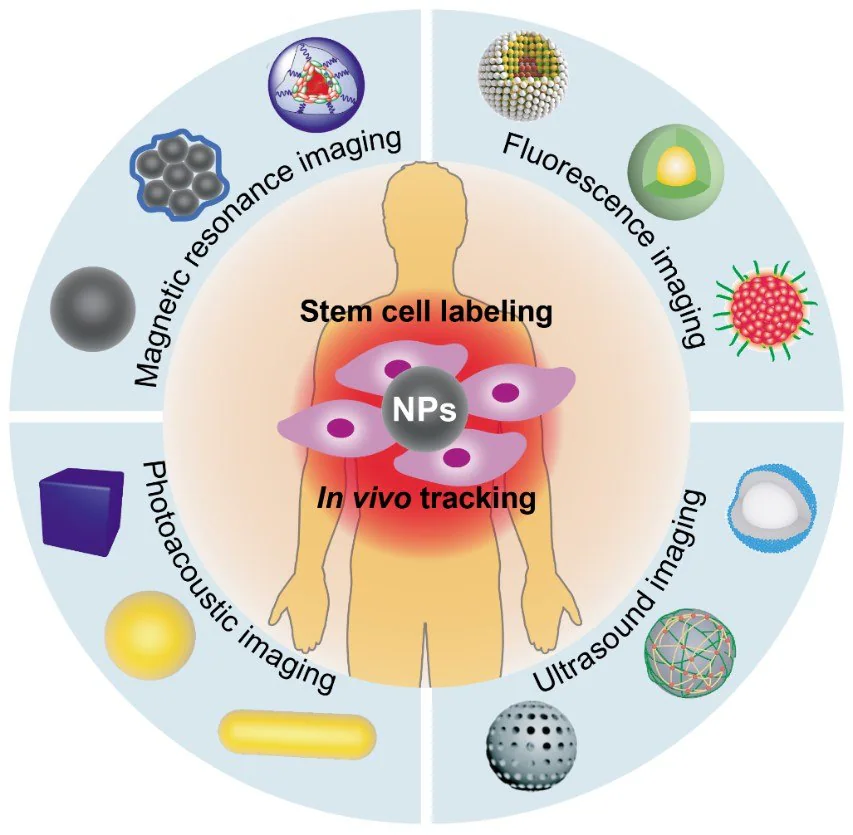
Figure 1. Various contract nanoagents designed for magnetic resonance imaging, fluorescence imaging, ultrasound imaging, and photoacoustic imaging modalities in stem cell tracking in vivo. Image Credit: Merck
Magnetic Resonance Imaging
The preferred method for in vivo stem cell imaging is MRI, owing to its distinctive attributes such as unrestricted penetration depth, exceptional spatial (ranging from 40 to 100 µm), and temporal (ranging from minutes to hours) resolution, and safe operational nature.1
MRI operates in two main categories: T1/T1* and T2/T2*-weighted MRI. These categories are determined by the relaxation time of the longitudinal (T1) and transverse (T2) components of the magnetization vector towards equilibrium below the applied magnetic field.
MRI contrast agents, such as gadolinium (III) chelates and superparamagnetic iron oxide nanoparticles (SIONPs), are introduced to heighten resolution and amplify signal intensity by influencing the relaxation times of nearby water protons.2
Gadolinium (III) chelates are efficient T1 contrast agents as they accelerate the longitudinal relaxation rate (T1), thereby enhancing positive contrast in T1-weighted MRI sequences.
SIONPs function as T2 contrast agents and have found widespread application in MRI-based cell tracking by producing negative contrast through the reduction of T2/T2* relaxation times.
The subsequent section reviews nanoparticle-based contrast agents that have demonstrated clinical or commercial validation for their utility in stem cell tracking applications.
Nanoparticle-based stem cell labeling agents necessitate specific criteria: colloidal stability, non-toxicity, strong magnetism, and efficient labeling. Achieving colloidal stability in aqueous solutions typically involves utilizing hydrophilic polymer coatings like chitosan, dextran, and PEG, among others.
A preclinical study by Margarita Gutova et al. demonstrated the transplantation of neural stem cells (NSCs) labeled with dextran-coated ferumoxytol (FDA-approved SIONPs) into patients with brain tumors.3 The distribution of NSCs was consistently monitored over 12 weeks at various intervals, followed by surveillance serial MRI scans.3
Enhanced labeling efficiency can be achieved by modifying particle surfaces to carry a positive charge or specific ligands.
For instance, compared to ferumoxytol, self-assembling ferumoxytol nano-complexes altered with heparin and protamine sulfate—which reversed the original particle's negative charge—exhibited increased labeling efficiency and a threefold rise in T2 relaxivity.
This modification approach demonstrated in vivo MRI detection of a minimum of 1000 HPF-labeled cells implanted within rat brains.4
Clinically approved Gd-DTPA enclosed within cationic liposomes ensured highly efficient uptake of Gd and exceptional intracellular retention in mesenchymal stem cells.
The Gd-DTPA-liposomes complex contrast agent rendered 500000 labeled stem cells distinctly visible for a minimum of two weeks on a 3.0 T clinical scanner, effectively overcoming the relatively low MRI sensitivity of Gd-DTPA.5
Monitoring the migration and survival of stem cells is crucial for both therapeutic efficacy and safety evaluations.
For investigating stem cell migration, Lili Jiang et al. observed that T2*-weighted MRI successfully tracked the migration of implanted pluripotent stem cells labeled with SIONPs (from Merck KGaA, Darmstadt, Germany) from the injection site to the injured brain areas for more than four weeks.6
In assessing cell survival, Ashok J. Theruvath et al. observed significant changes in ferumoxytol-labeled apoptotic matrix-associated stem cell implants (MASIs), indicating a substantial loss of iron signals and an extended T2 relaxation time, which persisted up to two weeks post-implantation during a cartilage repair process.7
When coupled with histopathologic examination, a ferumoxytol-based contrast agent could serve as an indicator distinguishing between living and deceased stem cells.
The duration for tracking stem cells varies depending on specific tissues, organs, and diseases, spanning from several days to months. An effective strategy for in vivo stem cell tracking involves evaluating cell distribution, migration, and differentiation, as well as assessing the efficacy and safety of cell implantation.
Despite the rapid advancements in nanoparticle-based MRI for stem cell tracking, certain challenges persist. These include imaging the differentiation and functionality of stem cells, integrating MRI contrast agents with reporter genes, and combining MRI with other noninvasive imaging tools.
Fluorescence Imaging
Fluorescence imaging, a traditional optical imaging method, is affordable and highly sensitive, but its effectiveness is limited by poor tissue penetration (<1 cm) in comparison to MRI technology.
One potential solution involves fine-tuning fluorophores or fluorescent proteins to be responsive within the near-infrared (NIR) range, enabling deeper tissue penetration. However, challenges like severe photo-bleaching and light scattering restrict tracking efficiency and duration.8
The evolution of fluorescent nanoparticles (NPs) has significantly enhanced the capability of fluorescent imaging for long-term stem cell tracing in vivo.
Quantum dots (QDs), a set of classic inorganic semiconductor NPs used for cell labeling,9 rely on the transition behaviors of excited electrons across various energy levels. These transitions can be adjusted by manipulating material components or compositions, enabling the realization of NIR emission.
For instance, CdSe/ZnS core/shell structures were developed specifically to label and track adipose tissue-derived stem cells (ASCs) in C57BL/6 mice models using NIR emission.10
Modifying the bandgap of materials significantly enhances the optical properties of QDs.11
Wang et al. coupled NIR-II fluorescence QDs of Ag2S with the traditional bioluminescence red firefly luciferase (RFLuc) to label human mesenchymal stem cells (hMSCs).
Using a wide spectrum spanning from 400 to 1700 nm, the researchers investigated the dynamic tracking of survival and osteogenic differentiation of the transplanted hMSCs in a mouse model with calvarial defects.12
Up-conversion nanoparticles (UCNPs) have also been employed to label and track mouse MSCs. UCNPs utilize the anti-Stokes process, absorbing multiple NIR photons to generate a single short-wavelength photon.13,14
Despite this, rare earth metal ions doped in UCNPs and heavy metal ions in QDs pose potential safety risks for tracing clinical stem cells. Aggregation-induced emission (AIE) fluorophores offer a secure method to track stem cells, boasting excellent photostability in comparison to inorganic NPs.15-18
AIE fluorophores remain non-emissive when dispersed but emit robust fluorescence when in an aggregated state due to limited intramolecular rotation, unlike aggregation caused by quenching (ACQ). This unique property makes Dots or AIE NPs present enduring and robust fluorescent signals.
For example, AIE Dots derived from tetraphenyl ethylene have demonstrated the ability to trace the journey of adipose-derived stem cells (ADSCs) over an extended period, outperforming green fluorescent proteins (FPs) and other bioluminescent molecules.18
It is also easy to adjust the AIE monomer for NIR or even NIR-II emission. The advancement of AIE Dots is an encouraging option for in vivo stem cell tracking, offering prolonged stability and reliability.
Ultrasound Imaging
Due to its remarkable temporal and spatial resolution and substantial tissue penetration depth, ultrasound imaging stands as a potent method for noninvasive and long-term cell tracking in stem cell therapies.19
However, its effectiveness is hindered by the limited contrast between implanted cells and neighboring soft tissues. To address this, ultrasound contrast agents (UCAs), a type of echogenic material, are deployed in clinical settings to enhance contrast and amplify detection signals.20
Traditional UCAs are micro-sized gas-filled bubbles, made of a bioinert heavy gas enveloped by stabilizing shells like proteins, lipids, and biocompatible polymers.21 The potential of these microbubbles for tracking stem cells is curbed by their inadequate structural stability, big microscale size, and short half-life.
Recent research has directed its attention toward scaling down UCAs, leading to the development of nanoscale UCAs such as nanobubbles, silica nanoparticles, and nanotubes tailored for ultrasound imaging.22
Achieving stability in the shell structure is crucial for nanobubbles. Leon et al. described a highly stable nanobubble type by employing a bilayer shell design with varying elastic properties akin to bacterial cell envelopes.23
These ultrastable nanobubbles exhibit minimal signal loss under continuous ultrasound exposure in vitro and boast a prolonged lifespan when tested in vivo. However, due to their small size, nanobubbles might lack sufficient ability to efficiently scatter ultrasonic waves.
To address this, a "small to large" transformation approach has been proposed. This strategy involves nanoscale UCAs transforming into microbubbles upon exposure to ultrasound, thereby amplifying the resulting echo signals.24 Gas-generating nanoparticles exemplify this concept.25,26
Min et al. reported a carbonate copolymer nanoparticle featuring a distinctive gas-generating mechanism. These nanoparticles undergo hydrolysis, yielding microscale CO2 bubbles that effectively absorb ultrasonic energy.27
Silica nanoparticles and similar glass-based nanomaterials significantly boost ultrasound signals due to tissue irregularities caused by the rigid characteristics of these nanoparticles, creating high impedance differences at tissue interfaces.28
Chen et al. detailed an innovative exosome-like silica (ELS) nanoparticle with high ultrasound impedance mismatches designed specifically for stem cell labeling and tracking using ultrasound.29
The researchers found that the nanoparticles' discoid shape, coupled with a positive charge, facilitated cellular uptake, and inherently heightened echogenicity. Silica nanoparticles with varied structures, like hollow and mesoporous configurations, also exhibited substantial ultrasound contrast.30
These silica-based nanoparticles show promise as UCAs for real-time stem cell tracking through ultrasound imaging due to their relatively robust structural integrity, low toxicity, and adaptable size and structure.
Photoacoustic Imaging
Biosafety and precise imaging are crucial for tracking cells in vivo over extended periods. PAI emerges as a promising biomedical imaging technique, based on the principle of the photoacoustic effect.
When a pulsed laser is introduced, it generates heat. The intermittent heat results in expansion, which is identified by PAI as a mechanical wave. This imaging method couples the strong contrast of optical imaging with the deep tissue penetration capabilities of ultrasonic imaging, offering real-time and high-resolution data in vivo.
PAI contrast nano agents are extensively used in vivo for imaging stem cells due to their exceptional capacity for photothermal conversion and the nanoparticles' biocompatibility
Various PAI contrast nano agents have been developed to absorb light across a spectrum, stretching from visible light to the NIR II region. This aims to minimize light absorbance and scattering within tissues.
Tracking methods that solely provide physical information, such as the whereabouts of labeled stem cells, do not meet the demands of studying stem cell engraftment. There is a pressing need for new platforms that can indicate real-time cell function and viability, crucial for clinical applications.
By focusing on the design of contrast nanoagents, new studies of PAI-based stem cell tracking were outlined in the following aspects: shape, component, size, and surface modification, shedding light on the essential factors that require consideration in the design of PAI contrast agents (Table 1).
Gold nanoparticles stand out among the various PAI contrast agents due to their notable advantages, including outstanding photothermal conversion efficiency, stable imaging capabilities, and high biosafety. The inert feature of gold nanoparticles has little effect on cell function.32
The Suggs group employed gold nanospheres of different sizes (20 nm, 40 nm, and 60 nm) in vivo to label and monitor MSCs.33
This study demonstrated the possibility of loading gold nanoparticles into MSCs for imaging purposes while preserving cell functionality post-loading. The research showed that gold nanoparticles remained detectable within stem cells for up to fourteen days, highlighting the potential for long-term and noninvasive cell tracking via PAI.
Anisotropic gold nanoparticles exhibit superior photothermal conversion efficiency. Jokerst et al. highlighted that applying a silica coating notably boosted the uptake rate of gold nanorods into stem cells by up to five times, achieving a minimum detection limit of 100000 labeled cells in vivo.34
The intricate transplant microenvironment in vivo poses challenges. Ricles et al. utilized a dual gold nanoparticle system, comprising gold nanorods and gold nanospheres, to track transplanted stem cells and image infiltrated macrophages.35
These studies introduced a novel approach to differentiate delivered stem cells from infiltrating immune cells, offering insights into the mechanisms of injury healing. Researchers also combined PAI with other imaging techniques to enhance tracking accuracy.
Nam et al. merged PAI with ultrasound imaging to monitor MSCs labeled with citrate-stabilized gold nanospheres.36 This combined approach offered both morphological insights through ultrasound imaging and functional information via photoacoustic imaging, allowing for spatial visualization of labeled MSCs.
Qiao et al. employed magnetic resonance and PAI techniques to track iron oxide (IO)@Au core-shell structure-labeled MSCs for imaging brain tumors.
This system exhibited potential for mapping cell trajectories and visualizing stem cell migration toward brain tumors in real time.37 Additionally, nanoparticles with NIR absorption capabilities were developed for stem cell tracking via PAI due to the superior penetration depth of NIR light.
Kim et al. utilized Prussian blue nanoparticles with robust light absorption at 740 nm for tracking stem cells.38
In vivo, these nanoagents demonstrated detection limits of 200 cells/μL, enabling monitoring for up to fourteen days post-injection, attributed to the excellent bio-stability and NIR-I detectability. Yin et al. developed a NIR-II organic semiconductor polymer specifically for stem cell tracking via PAI.39
The researchers observed a significant 40.6-fold and 21.7-fold enhancement in subcutaneous and brain imaging, respectively, primarily due to the deep tissue penetration of NIR-II light.
To monitor stem cell activity post-transplantation, Dhada et al. engineered a ROS-sensitive dye (R775c) coated onto gold nanorods.
The strategy leveraged the fact that stem cells release ROS to degrade dying cells in vivo, enabling the measurement of stem cell viability. Consequently, they could simultaneously visualize cell viability and location in vivo using PAI.40
Table 1. The design of nanoparticle-based contrast agents for PAI. Source: Merck
Year |
Shape |
Surface Modification |
Charge |
Size |
Concentration |
Tracking Period |
2011 [33] |
Gold nanosphere |
poly-L-lysine |
+30 mV |
20, 40, 60 nm |
1012 nanoparticles/mL |
14 days |
2012 [34] |
Gold nanorod |
silica |
NA |
83 x 64 nm |
4 x 1013 nanoparticles/mL |
4 days |
2014 [35] |
Dual gold nanoparticle system (rods and spheres) |
mPEG-SH for spheres; poly-L-lysine for silica rods |
-8,41 mV of spheres, +26
mV of rods |
50 nm; 56 x 16 nm |
Both 1012 nanoparticles/mL |
7 days |
2011 [36] |
Gold nanosphere |
citrate |
NA |
20 nm |
1.13 x 1011 nanoparticles/mL |
in vitro study |
2018 [37] |
IO@Au core-shell structure |
mPEG-NH2 |
NA |
82 nm |
4 µg/mL |
3 days |
2017 [38] |
Prussian blue nanoparticles |
Poly(L-lysine) |
+33.9 mV |
133.8 nm |
50 µg/mL |
14 days |
2018 [39] |
Organic semiconducting polymer |
Poly(L-lysine) |
+42 mV |
99.9 nm |
50 µg/mL |
14 days |
2019 [40] |
Silica coated R775c@gold nanorod |
poly-D-lysine |
+8mV |
450 nm |
20 µg/mL |
10 days |
Table 2. Comparison of different imaging modalities. Source: Merck
Imaging Modality |
Magnetic Resonance Imaging |
Fluorescence Imaging |
Ultrasound Imaging |
Photoacoustic Imaging |
Advantages |
Deep penetration; High spatial resolution; Safety |
High sensitivity/resolution; Highly developed device |
Safe, deep penetration; Real-time dynamic imaging |
High resolution; 3-dimensional imaging |
Disadvantages |
Signal decays as the material degrades; Signal intensity is not linearly proportional to its concentration |
Light bleaching; Superficial imaging; Pedigree tracer requires transfection and has limited optical penetration |
Short life and large size of contrast agents |
The blood signals interference; Laser penetrable imaging |
Nano agents offer key advantages over organic molecules in PAI imaging, primarily in terms of photostability, water solubility, and biocompatibility.
The limitations of PAI hinge on the penetration depth and photothermal conversion efficiency of contrast nano agents. Therefore, future advancements in PAI imaging are likely to focus on developing nanomaterials with enhanced NIR-II responsiveness and improved biocompatibility.
Conclusion and Outlook
Advancing stem cell-based therapies heavily relies on the progress of stem cell tracking techniques. Current imaging technologies present both strengths and limitations (Table 2).
Consequently, the choice of imaging modalities depends on specific tissue requirements and imaging depth.
A range of nanoparticles, including iron oxide nanoparticles, quantum dots, aggregative-induced emission nanoparticles, silica nanoparticles, and gold nanoparticles, have been employed for stem cell tracing due to their distinct physical-chemical properties. These properties enhance imaging signals upon irradiation.
By elaborately designing the structures and compositions, nanoparticles play a pivotal role in improving the performance of existing imaging modalities concerning imaging resolution, stability, and lifespan. However, tracking stem cells in vivo remains a significant challenge in the biomedical field.
The amplitude of the image signal typically correlates linearly with contrast nanoagent concentration, but as contrast agents within cells dilute through cell division, challenges arise. This fuels the necessity for a new generation of contrast agents that resist dilution or prompt cells to self-synthesize these agents, addressing this issue.
Additionally, apart from cell division, studies indicate that nanoparticle levels within cells achieve equilibrium via endocytosis and exocytosis, potentially leading to false positive detections in vivo.
Moreover, few contrast agents can capture a cell's live state and normal functional abilities, severely limiting the exploration of stem cell therapy mechanisms. Therefore, concerted efforts are crucial to design multifunctional contrast agents that address these concerns.
Lastly, the advancement of both imaging devices (the "hardware") and imaging contrast agents (the "software") is urgently needed. This development aims to enable precise, long-term, and dynamic tracking of transplanted stem cells, a critical aspect of the field.
References and Further Reading
- Barrow M, Taylor A, Murray P, Rosseinsky MJ, Adams DJ. 2015. Design considerations for the synthesis of polymer coated iron oxide nanoparticles for stem cell labelling and tracking using MRI. Chem. Soc. Rev.. 44(19):6733-6748. https://doi.org/10.1039/c5cs00331h
- Zhang B, Yan W, Zhu Y, Yang W, Le W, Chen B, Zhu R, Cheng L. 2018. Nanomaterials in Neural-Stem-Cell-Mediated Regenerative Medicine: Imaging and Treatment of Neurological Diseases. Adv. Mater.. 30(17):1705694. https://doi.org/10.1002/adma.201705694
- Gutova M, Frank JA, D'Apuzzo M, Khankaldyyan V, Gilchrist MM, Annala AJ, Metz MZ, Abramyants Y, Herrmann KA, Ghoda LY, et al. 2013. Magnetic Resonance Imaging Tracking of Ferumoxytol-Labeled Human Neural Stem Cells: Studies Leading to Clinical Use. 2(10):766-775. https://doi.org/10.5966/sctm.2013-0049
- Thu MS, Bryant LH, Coppola T, Jordan EK, Budde MD, Lewis BK, Chaudhry A, Ren J, Varma NRS, Arbab AS, et al. 2012. Self-assembling nanocomplexes by combining ferumoxytol, heparin and protamine for cell tracking by magnetic resonance imaging. Nat Med. 18(3):463-467. https://doi.org/10.1038/nm.2666
- Guenoun J, Koning GA, Doeswijk G, Bosman L, Wielopolski PA, Krestin GP, Bernsen MR. 2012. Cationic Gd-DTPA Liposomes for Highly Efficient Labeling of Mesenchymal Stem Cells and Cell Tracking with MRI. Cell Transplant. 21(1):191-205. https://doi.org/10.3727/096368911x593118
- Jiang L, Li R, Tang H, Zhong J, Sun H, Tang W, Wang H, Zhu J. 2019. MRI Tracking of iPS Cells-Induced Neural Stem Cells in Traumatic Brain Injury Rats. Cell Transplant. 28(6):747-755. https://doi.org/10.1177/0963689718819994
- Theruvath AJ, Nejadnik H, Lenkov O, Yerneni K, Li K, Kuntz L, Wolterman C, Tuebel J, Burgkart R, Liang T, et al. 2019. Tracking Stem Cell Implants in Cartilage Defects of Minipigs by Using Ferumoxytol-enhanced MRI. Radiology. 292(1):129-137. https://doi.org/10.1148/radiol.2019182176
- Kircher MF, Gambhir SS, Grimm J. 2011. Noninvasive cell-tracking methods. Nat Rev Clin Oncol. 8(11):677-688. https://doi.org/10.1038/nrclinonc.2011.141
- Yu Y, Feng C, Hong Y, Liu J, Chen S, Ng KM, Luo KQ, Tang BZ. 2011. Cytophilic Fluorescent Bioprobes for Long-Term Cell Tracking. Adv. Mater.. 23(29):3298-3302. https://doi.org/10.1002/adma.201101714
- Yukawa H, Kagami Y, Watanabe M, Oishi K, Miyamoto Y, Okamoto Y, Tokeshi M, Kaji N, Noguchi H, Ono K, et al. 2010. Quantum dots labeling using octa-arginine peptides for imaging of adipose tissue-derived stem cells. Biomaterials. 31(14):4094-4103. https://doi.org/10.1016/j.biomaterials.2010.01.134
- Yang Y, Chen J, Shang X, Feng Z, Chen C, Lu J, Cai J, Chen Y, Zhang J, Hao Y, et al. 2019. Visualizing the Fate of Intra?Articular Injected Mesenchymal Stem Cells In Vivo in the Second Near?Infrared Window for the Effective Treatment of Supraspinatus Tendon Tears. Adv. Sci.. 6(19):1901018. https://doi.org/10.1002/advs.201901018
- Huang D, Lin S, Wang Q, Zhang Y, Li C, Ji R, Wang M, Chen G, Wang Q. 2019. An NIR-II Fluorescence/Dual Bioluminescence Multiplexed Imaging for In Vivo Visualizing the Location, Survival, and Differentiation of Transplanted Stem Cells. Adv. Funct. Mater.. 29(2):1806546. https://doi.org/10.1002/adfm.201806546
- Wang C, Cheng L, Xu H, Liu Z. 2012. Towards whole-body imaging at the single cell level using ultra-sensitive stem cell labeling with oligo-arginine modified upconversion nanoparticles. Biomaterials. 33(19):4872-4881. https://doi.org/10.1016/j.biomaterials.2012.03.047
- Wilhelm S. 2017. Perspectives for Upconverting Nanoparticles. ACS Nano. 11(11):10644-10653. https://doi.org/10.1021/acsnano.7b07120
- Li K, Zhu Z, Cai P, Liu R, Tomczak N, Ding D, Liu J, Qin W, Zhao Z, Hu Y, et al. 2013. Organic Dots with Aggregation-Induced Emission (AIE Dots) Characteristics for Dual-Color Cell Tracing. Chem. Mater.. 25(21):4181-4187. https://doi.org/10.1021/cm401709d
- Li K, Yamamoto M, Chan SJ, Chiam MY, Qin W, Wong PTH, Yim EKF, Tang BZ, Liu B. 2014. Organic nanoparticles with aggregation-induced emission for tracking bone marrow stromal cells in the rat ischemic stroke model. Chem. Commun.. 50(96):15136-15139. https://doi.org/10.1039/c4cc06921h
- Gao M, Chen J, Lin G, Li S, Wang L, Qin A, Zhao Z, Ren L, Wang Y, Tang BZ. 2016. Long-Term Tracking of the Osteogenic Differentiation of Mouse BMSCs by Aggregation-Induced Emission Nanoparticles. ACS Appl. Mater. Interfaces. 8(28):17878-17884. https://doi.org/10.1021/acsami.6b05471
- Ding D, Mao D, Li K, Wang X, Qin W, Liu R, Chiam DS, Tomczak N, Yang Z, Tang BZ, et al. 2014. Precise and Long-Term Tracking of Adipose-Derived Stem Cells and Their Regenerative CapacityviaSuperb Bright and Stable Organic Nanodots. ACS Nano. 8(12):12620-12631. https://doi.org/10.1021/nn505554y
- Abou-Elkacem L, Bachawal SV, Willmann JK. 2015. Ultrasound molecular imaging: Moving toward clinical translation. European Journal of Radiology. 84(9):1685-1693. https://doi.org/10.1016/j.ejrad.2015.03.016
- Son S, Min HS, You DG, Kim BS, Kwon IC. 2014. Echogenic nanoparticles for ultrasound technologies: Evolution from diagnostic imaging modality to multimodal theranostic agent. Nano Today. 9(4):525-540. https://doi.org/10.1016/j.nantod.2014.06.002
- Li Y, Chen Y, Du M, Chen Z. 2018. Ultrasound Technology for Molecular Imaging: From Contrast Agents to Multimodal Imaging. ACS Biomater. Sci. Eng.. 4(8):2716-2728. https://doi.org/10.1021/acsbiomaterials.8b00421
- Smith BR, Gambhir SS. 2017. Nanomaterials for In Vivo Imaging. Chem. Rev.. 117(3):901-986. https://doi.org/10.1021/acs.chemrev.6b00073
- Leon A, Perera R, Hernandez C, Cooley M, Jung O, Jeganathan S, Abenojar E, Fishbein G, Sojahrood AJ, Emerson CC, et al. 2019. Contrast enhanced ultrasound imaging by nature-inspired ultrastable echogenic nanobubbles. Nanoscale. 11(33):15647-15658. https://doi.org/10.1039/c9nr04828f
- Liu J, Shang T, Wang F, Cao Y, Hao L, Ren J, Ran H, Wang Z, Li P, Du Z. 2019. Low-intensity focused ultrasound (LIFU)-induced acoustic droplet vaporization in phase-transition perfluoropentane nanodroplets modified by folate for ultrasound molecular imaging. IJN. Volume 12911-923. https://doi.org/10.2147/ijn.s122667
- Sun I, Emelianov S. Gas-generating nanoparticles for contrast-enhanced ultrasound imaging. Nanoscale. 11(35):16235-16240. https://doi.org/10.1039/c9nr04471j
- Gao S, Wang G, Qin Z, Wang X, Zhao G, Ma Q, Zhu L. 2017. Oxygen-generating hybrid nanoparticles to enhance fluorescent/photoacoustic/ultrasound imaging guided tumor photodynamic therapy. Biomaterials. 112324-335. https://doi.org/10.1016/j.biomaterials.2016.10.030
- Min HS, Son S, You DG, Lee TW, Lee J, Lee S, Yhee JY, Lee J, Han MH, Park JH, et al. 2016. Chemical gas-generating nanoparticles for tumor-targeted ultrasound imaging and ultrasound-triggered drug delivery. Biomaterials. 10857-70. https://doi.org/10.1016/j.biomaterials.2016.08.049
- Ma M, Shu Y, Tang Y, Chen H. 2020. Multifaceted application of nanoparticle-based labeling strategies for stem cell therapy. Nano Today. 34100897. https://doi.org/10.1016/j.nantod.2020.100897
- Chen F, Ma M, Wang J, Wang F, Chern S, Zhao ER, Jhunjhunwala A, Darmadi S, Chen H, Jokerst JV. Exosome-like silica nanoparticles: a novel ultrasound contrast agent for stem cell imaging. Nanoscale. 9(1):402-411. https://doi.org/10.1039/c6nr08177k
- Qi S, Zhang P, Ma M, Yao M, Wu J, Mäkilä E, Salonen J, Ruskoaho H, Xu Y, Santos HA, et al. 2019. Cellular Internalization-Induced Aggregation of Porous Silicon Nanoparticles for Ultrasound Imaging and Protein-Mediated Protection of Stem Cells. Small. 15(1):1804332. https://doi.org/10.1002/smll.201804332
- Wang LV, Hu S. 2012. Photoacoustic Tomography: In Vivo Imaging from Organelles to Organs. Science. 335(6075):1458-1462. https://doi.org/10.1126/science.1216210
- Ferreira L. 2009. Nanoparticles as tools to study and control stem cells. J. Cell. Biochem.. 108(4):746-752. https://doi.org/10.1002/jcb.22303
- Ricles L, Nam, Sokolov K, Emelianov S, Suggs L. Function of mesenchymal stem cells following loading of gold nanotracers. IJN.407. https://doi.org/10.2147/ijn.s16354
- Jokerst JV, Thangaraj M, Kempen PJ, Sinclair R, Gambhir SS. 2012. Photoacoustic Imaging of Mesenchymal Stem Cells in Living Mice via Silica-Coated Gold Nanorods. ACS Nano. 6(7):5920-5930. https://doi.org/10.1021/nn302042y
- Ricles LM, Nam SY, Treviño EA, Emelianov SY, Suggs LJ. A dual gold nanoparticle system for mesenchymal stem cell tracking. J. Mater. Chem. B. 2(46):8220-8230. https://doi.org/10.1039/c4tb00975d
- Nam SY, Ricles LM, Sokolov K, Suggs LJ, Emelianov SY. 2011. Ultrasound and photoacoustic imaging to monitor mesenchymal stem cells labeled with gold nanoparticles. https://doi.org/10.1117/12.875262
- Qiao Y, Gumin J, MacLellan CJ, Gao F, Bouchard R, Lang FF, Stafford RJ, Melancon MP. 2018. Magnetic resonance and photoacoustic imaging of brain tumor mediated by mesenchymal stem cell labeled with multifunctional nanoparticle introduced via carotid artery injection. Nanotechnology. 29(16):165101. https://doi.org/10.1088/1361-6528/aaaf16
- Kim T, Lemaster JE, Chen F, Li J, Jokerst JV. 2017. Photoacoustic Imaging of Human Mesenchymal Stem Cells Labeled with Prussian Blue?Poly(l-lysine) Nanocomplexes. ACS Nano. 11(9):9022-9032. https://doi.org/10.1021/acsnano.7b03519
- Yin C, Wen G, Liu C, Yang B, Lin S, Huang J, Zhao P, Wong SHD, Zhang K, Chen X, et al. 2018. Organic Semiconducting Polymer Nanoparticles for Photoacoustic Labeling and Tracking of Stem Cells in the Second Near-Infrared Window. ACS Nano. 12(12):12201-12211. https://doi.org/10.1021/acsnano.8b05906
- Dhada KS, Hernandez DS, Suggs LJ. 2019. In Vivo Photoacoustic Tracking of Mesenchymal Stem Cell Viability. ACS Nano. 13(7):7791-7799. https://doi.org/10.1021/acsnano.9b01802
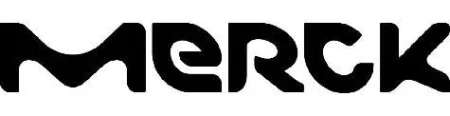
This information has been sourced, reviewed, and adapted from materials provided by Merck.
For more information on this source, please visit Merck.