Sponsored by MerckReviewed by Emily MageeJan 23 2024
In recent years, fluorescence quenching microscopy (FQM)1-3 has emerged as a viable technique that allows for the swift, cost-effective, and accurate imaging of two-dimensional (2D) materials like graphene-based sheets (Product No. 763713, 794341, and 777684) and MoS2 (Product No. 234842 and 69860).
Graphene and other emerging 2D materials have attracted a great deal of interest in recent years due to their novel properties and exciting applications,4 but the characterization of these materials has remained challenging.
In conventional fluorescence microscopy, imaging is enabled by fluorescent labeling, where excitation makes the targets lighten up against a dark background. However, this method struggles when faced with strong fluorescence quenchers like graphite and graphene-based sheets.1,5,6
Contrastingly, FQM-based techniques use a reverse approach, where a fluorescent layer is applied to cover both the targets and their surrounding area. Upon excitation, graphene-based sheets appear dark against a bright background.
The long-range fluorescence quenching capability of 2D materials1–3,5 also allows differentiation between overlapping layers, wrinkles, and folds, as they appear darker than single layers.
Therefore, FQM is capable of producing crisp images with contrast and layer resolution comparable to those obtained from atomic force microscopy (AFM) and scanning electron microscopy (SEM).
Another advantage of FQM is its versatility across various formats: it is capable of imaging 2D sheets on arbitrary substrates as well as in solution.
Basic Principles of Imaging 2D Materials by FQM
Graphene-based sheets quench dye molecules' fluorescence through long-range energy transfer via dipole-dipole interactions or short-range electron/charge transfer. The latter relies on orbital overlap and operates within limited molecular contact distances.
In contrast, dipole-dipole interactions can extend over much longer distances, even through empty or occupied space.7
While both mechanisms result in fluorescence quenching, only the long-range mechanism, essentially Forster resonance energy transfer (FRET), is suitable for Fluorescence Quenching Microscopy (FQM). This is because it allows for contrast differentiation between varying numbers of layers.1–3,8
In FQM measurements, signal contrast is generated through the difference in fluorescence quenching between the sample of interest and the substrate. This contrast mechanism is quite generic and applicable across various materials.
Due to its diffraction-limited lateral resolution when used with a standard optical microscope, FQM excels in imaging objects with micron-scale lateral dimensions like graphene sheets, graphene oxide, and other 2D material flakes.
Methods and Procedures of FQM
FQM simplifies sample preparation by spin-coating a dye-infused polymer layer onto a substrate. This process involves adding a polymer to create a uniform thin film, onto which a fluorescent dye is dispersed for imaging purposes.
Due to the broadband quenching capability of graphene-based sheets, FQM is not limited by the wavelength of illumination and can be performed using a wide range of fluorescent materials. Consequently, a multitude of dye/polymer combinations can be employed based on their suitability for different solvents and their capacity to form thin films.
For instance, fluorescein (Product No. 46955)/polyvinylpyrrolidone (Product No. 234257, 856568, and 437190) (FL/PVP) can be used in water or ethanol.
Similarly, 4-(dicyanomethylene)-2-methyl-6-(4-dimethylaminostyryl)-4H-pyran (Product No. 410497)/poly(methyl methacrylate) (Product No. 182230, 445746, etc.) (DCM/PMMA) is applicable in organic solvents like chloroform (Product No. 437581, etc.), toluene (Product No. 179418, etc.), and anisole (Product No. 123226 and 296295).
The fluorescent layer can be either on top of the sample or beneath it, and the thickness of the dye/polymer layer influences FQM image contrast. Dye layer thickness in the range of tens of nanometers has been shown to produce the highest quality FQM images, achieving high contrast and the ability to resolve different numbers of layers.
An FQM image of graphene oxide (GO) sheets deposited on a glass coverslip taken with a digital camera directly through an eyepiece of a fluorescence microscope represents the view under FQM from the perspective of the naked eye. It is important to note that if the substrate itself is fluorescent, this auto-fluorescence can be utilized to conduct FQM directly, eliminating the need for an additional dye layer.
FQM holds distinct advantages over three common microscopy methods—reflectance-mode optical microscopy, atomic force microscopy (AFM), and scanning electron microscopy (SEM)—used for imaging 2D materials.
These traditional techniques are constrained to 2D sheets on specific substrates. Reflectance-mode optical microscopy, for instance, relies on samples deposited on particular Si wafers for adequate contrast.
AFM, a low-throughput metrology method, is limited to imaging small sample areas on exceptionally smooth substrates at a molecular level. SEM requires sheets to be on conductive substrates and operates solely under vacuum.
In contrast, FQM is more adaptable, tolerating considerable surface roughness compared to AFM and reflectance spectroscopy. It functions effectively across a wide array of substrates, encompassing metal, glass, and plastic.
While previously it has been difficult to image graphene and related 2D materials on glass or plastic substrates, FQM finally allows for rapid microscopy of these 2D sheets using common chemicals and lab supplies.
Capabilities of FQM
Thanks to its user-friendly nature and affordability, FQM has gained traction among numerous research groups for characterizing graphene-based sheets. It is particularly adept at swiftly assessing sample or thin film morphology, a task that was previously reliant on SEM analysis.
Given its ability to produce high-quality images akin to SEM but on more cost-effective substrates like coverslips, FQM promises to replace SEM for routine imaging of many micron-sized sheets.
At Northwestern University, FQM has even found a place in an undergraduate lab module. Here, students employ the technique to explore how processing methods impact the microstructures of graphene-based sheets.
In FQM images of GO sheets deposited on glass coverslips by spin coating and Langmuir-Blodgett (LB) assembly,9 other than the obvious difference in coverage, the spin-coated sample contains a significantly higher fraction of small GO pieces than the LB sample. This is due to the size-dependent amphiphilicity of GO.10-12
Smaller sheets possess greater charge density, rendering them more hydrophilic and less likely to remain on the water surface during LB assembly.
FQM's abilities extend to directly visualizing GO sheets in water. An FQM image is able to distinctly show a GO sheet suspended in an aqueous fluorescein solution—a detail barely visible under transmission mode.
This feature facilitates real-time observation of how capillary action manipulates GO sheets during dewetting. This observation led to the discovery of crumpled, paper-ball-like graphene via an aerosol-based synthetic route.8,13
As mentioned earlier, FQM's applicability hinges on detecting differences in fluorescence quenching capabilities between the sample and substrate. Therefore, FQM can even visualize graphene deposited on metal surfaces, which themselves are strong quenchers.
For instance, graphene flakes grown on Cu foil (Product No. 773697) via chemical vapor deposition (CVD).3
FQM boasts another niche ability—it effortlessly generates contrast between graphene samples exhibiting varying degrees of graphitization. An FQM image is able to showcase a stark contrast between GO and reduced GO (r-GO), with r-GO exhibiting stronger fluorescence quenching due to its higher graphitic nature.1
This capability has proved invaluable for visualizing graphene patterns or circuits created by inscribing insulating sp3 domains on graphene sheets.14 Recently, FQM has also expanded its scope to visualize MoS2 sheets.3
Concerns have surfaced regarding the potential introduction of extra processing steps and contamination sources due to the dye/polymer coating used in FQM. However, for most routine sample testing needs, this is not a significant issue as the majority of samples do not tend to undergo further experimentation post-microscopy.
Even in cases involving subsequent experimentation on the same sample, integrating FQM into existing processing steps is feasible without introducing added complexity. It may even open up new capabilities.
For instance, a layer of PMMA is often applied to CVD-grown graphene samples to remove them from the metal foil substrate, which can be used to form the fluorescence layer for FQM.
Similarly, device fabrication often entails coating samples with a polymeric layer of photoresist or e-beam resist, which can also double up for FQM purposes.
Similarly, in device fabrication, samples are often coated with a polymeric layer of photoresist or e-beam resist, which can also be utilized for FQM. Interestingly, this allows sheet-specific projection lithography to first select a flake and then perform photolithography of the same flake under a fluorescence microscope.2
Finally, as the dye is dispersed within the polymer layer, only a limited number of dye molecules come into contact with the 2D sheets. At least in the FL/PVP system, the fluorescent layer can be conveniently removed by water and ethanol without disrupting or contaminating the underlying sheets.1
References
- Kim J, Cote LJ, Kim F, Huang J. 2010. Visualizing Graphene Based Sheets by Fluorescence Quenching Microscopy. J. Am. Chem. Soc.. 132(1):260-267. https://doi.org/10.1021/ja906730d
- Kim J, Kim F, Huang J. 2010. Seeing graphene-based sheets. Materials Today. 13(3):28-38. https://doi.org/10.1016/s1369-7021(10)70031-6
- Tan ATL, Kim J, Huang J, Li L, Huang J. 2013. Seeing Two-Dimensional Sheets on Arbitrary Substrates by Fluorescence Quenching Microscopy. Small.n/a-n/a. https://doi.org/10.1002/smll.201300049
- Butler SZ, Hollen SM, Cao L, Cui Y, Gupta JA, Gutiérrez HR, Heinz TF, Hong SS, Huang J, Ismach AF, et al. 2013. Progress, Challenges, and Opportunities in Two-Dimensional Materials Beyond Graphene. ACS Nano. 7(4):2898-2926. https://doi.org/10.1021/nn400280c
- Swathi RS, Sebastian KL. 2009. Long range resonance energy transfer from a dye molecule to graphene has (distance)?4 dependence. The Journal of Chemical Physics. 130(8):086101. https://doi.org/10.1063/1.3077292
- Kagan MR, McCreery RL. 1994. Reduction of Fluorescence Interference in Raman Spectroscopy via Analyte Adsorption on Graphitic Carbon. Anal. Chem.. 66(23):4159-4165. https://doi.org/10.1021/ac00095a008
- Nicholas Turro J, Ramamurthy V, Scaiano J. 2009. Principles of molecular photochemistry: An introduction. 495. University Science Books: Sausalito, Calif.:
- Luo J, Kim J, Huang J. 2013. Material Processing of Chemically Modified Graphene: Some Challenges and Solutions. Acc. Chem. Res.. 46(10):2225-2234. https://doi.org/10.1021/ar300180n
- Cote LJ, Kim F, Huang J. 2009. Langmuir?Blodgett Assembly of Graphite Oxide Single Layers. J. Am. Chem. Soc.. 131(3):1043-1049. https://doi.org/10.1021/ja806262m
- Kim F, Cote LJ, Huang J. 2010. Graphene Oxide: Surface Activity and Two-Dimensional Assembly. Adv. Mater.. 22(17):1954-1958. https://doi.org/10.1002/adma.200903932
- Kim J, Cote LJ, Kim F, Yuan W, Shull KR, Huang J. 2010. Graphene Oxide Sheets at Interfaces. J. Am. Chem. Soc.. 132(23):8180-8186. https://doi.org/10.1021/ja102777p
- Cote LJ, Kim J, Tung VC, Luo J, Kim F, Huang J. 2010. Graphene oxide as surfactant sheets. 83(1):95-110. https://doi.org/10.1351/pac-con-10-10-25
- Luo J, Jang HD, Sun T, Xiao L, He Z, Katsoulidis AP, Kanatzidis MG, Gibson JM, Huang J. 2011. Compression and Aggregation-Resistant Particles of Crumpled Soft Sheets. ACS Nano. 5(11):8943-8949. https://doi.org/10.1021/nn203115u
- Sun Z, Pint CL, Marcano DC, Zhang C, Yao J, Ruan G, Yan Z, Zhu Y, Hauge RH, Tour JM. 2011. Towards hybrid superlattices in graphene. Nat Commun. 2(1): https://doi.org/10.1038/ncomms1577
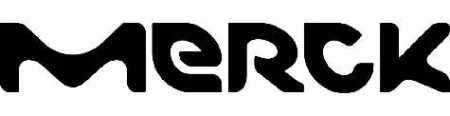
This information has been sourced, reviewed and adapted from materials provided by Merck.
For more information on this source, please visit Merck.