The field of tissue engineering aims to repair and regenerate damaged organs or tissues using ex vivo tissue constructs.
A key challenge lies in the design and fabrication of scaffolds that facilitate three-dimensional (3D) tissue growth or wound healing for clinical applications, such as aiding the preparation of bioartificial transplants.
Scaffolds such as these should be biocompatible for the adhesion of cells and should also have high porosity and sufficient pore size. These qualities are all required to enable the diffusion of oxygen and nutrients to cells throughout the entire structure.
Biodegradability is frequently required because some scaffolds should ideally be absorbed by the surrounding tissues at an appropriate rate without surgical removal.
Scaffolds should deliver the desired form, architecture, and mechanical stability to simulate and support the native tissue.
Visualizing the morphology and characterizing the microstructures of the designed scaffold in 3D, such as the pore size, porosity, and fiber morphology, is critical for the design and fabrication of tailored and application-optimized materials.
This article discusses the research published by C. S. de Oliveira et al.1 It presents the X-Ray microscopy (XRM) characterization of the crosslinked electrospun gelatin nanofibers that may be utilized as a nanofibrous scaffold for skin wound dressing and skin regeneration in medical care.
Cross-Linked Gelatin Nanofibrous Scaffold
Gelatin is a biodegradable and biocompatible natural biomaterial that delivers a suitable environment for the attachment and spreading of cells. This makes it an appropriate base material for tissue engineering.
When gelatin nanofibrous scaffolds were fabricated using the electrospinning method, the nanofibers exhibited a random, nonwoven architecture with interconnected pores, mimicking the extracellular matrix of the native tissues such as skin.1
To increase the stability of the gelatin mat, it was subjected to a formaldehyde-rich atmosphere for varying periods, leading to chemical cross-linking of the fibers and morphological changes of the mats due to fiber thickening.
3D Characterization of Cross-Linked Gelatin Nanofibrous Scaffold Using Phase-Contrast
The 3D characterization of the porous structure of the cross-linked gelatin scaffolds using X-Ray microscopy is complex due to the gelatin nanofibers consisting of elements with a low atomic number, meaning they provide extremely low X-Ray absorption-contrast.
However, visualizing the morphology and characterizing the sample features associated with the 3D structure of the gelatin scaffold, such as the porosity and fiber thickness in the volume, is vital for the design and fabrication of tailored and application-optimized materials.
The Zeiss Xradia 810 Ultra, a lab-based X-Ray microscope equipped with a chromium source (5.4 keV) and integrated phase-contrast technology, may be employed to improve the 3D visibility of nanostructures of biopolymers with low absorption contrast.
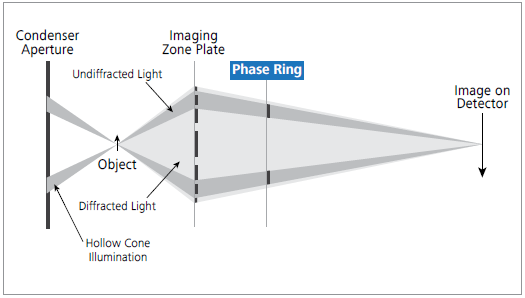
Figure 1. The X-Ray beam path of ZEISS Xradia Ultra 810. In a laboratory setup, a phase ring is inserted between the Fresnel zone plate objective and the detector for unique Zernike phase-contrast. Image Credit: Carl Zeiss Microscopy GmbH
C. S. de Oliveira et al. utilized the Xradia 810 Ultra to carry out Zernike phase-contrast imaging to visualize and characterize microstructures of electrospun nanofibrous scaffolds.
In the Xradia 810 Ultra, the sample is lit by an annular beam, and a phase ring is inserted in the beam path after the objective (see Figure 1).
This phase ring adjusts the phase of the background light relative to the light scattered by the specimen. The interference of the two beams in the detector plane translates phase shifts into intensity variations detected downstream.
All samples were scanned with 901 projection images (with a field-of-view of 64 μm2) obtained by the sample being rotated over 180°.
The exposure time was 30 seconds, and a detector binning of 1×1 was employed (with an isotropic voxel size of 64 nm3) for the thinner (pristine) samples. These samples were subsequently cross-linked for 60 minutes and 90 minutes.
To decrease the scan time while maintaining comparable X-Ray counts, an exposure time of 15 seconds was utilized for imaging the thicker samples, which were subsequently cross-linked for 120 minutes and 180 minutes, with a detector binning of 2×2.
The AMC (adaptive motion compensation) option provided by the software of Xradia 810 Ultra was employed to account for any sample drift. The AMC runs a quick scan after the main scan, using a few projections later utilized to rectify large sample drifts.
The volumetric reconstruction of the dataset was carried out with XRM Reconstructor software integrated into the Xradia 810 Ultra. Figure 2 displays the spatial distribution of the fibers in volumetric XRM images and their morphological changes with increasing cross-link duration.
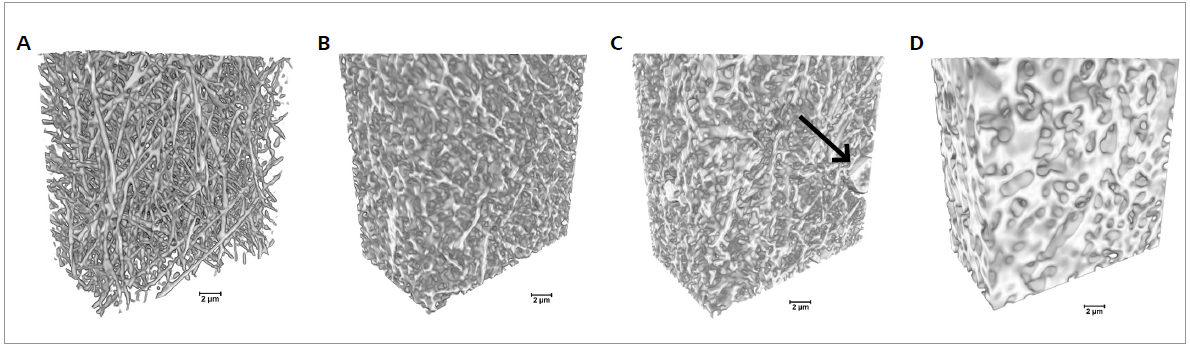
Figure 2. 3D imaging of electrospun gelatin fibers cross-linked for different times using Xradia 810 Ultra. The cross-linking duration increased from 0 min (pristine gelatin sample) to 60, 90, and 120 min from A to D, respectively. Arrow indicates a bead in the fibrous mats (scale bar: 2 μm). Image Credit: Carl Zeiss Microscopy GmbH
The 3D dataset allows the simple visualization of the presence and distribution of beads in the samples and the interaction with the surrounding fibers in the space.
The diameter distribution for the fibers that were estimated using the 3D dataset is displayed in Figure 3. Using the 3D XRM datasets, a trend of fiber diameter increase with increasing cross-linking time. The thin pristine gelatin fibers merge with the cross-linking.
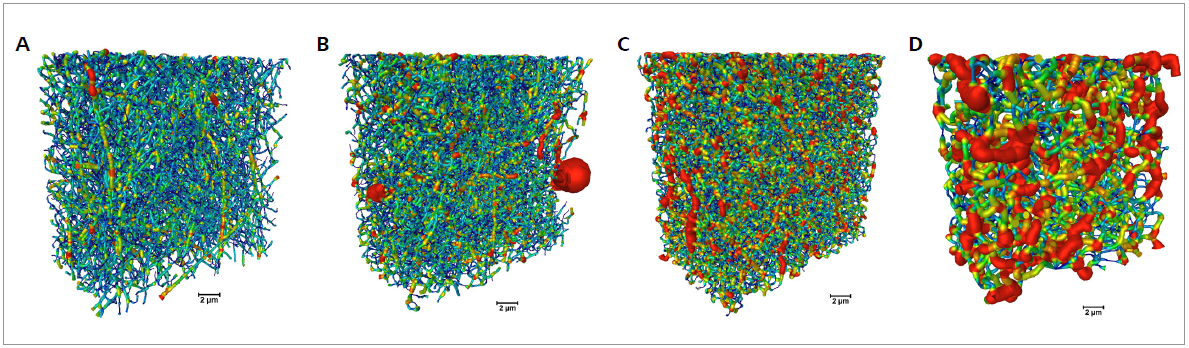
Figure 3. Fiber characterization for samples cross-linked for different times (0 to 120 min from A to D, respectively). The volumetric color-maps encode the thickness of the fibers, which is correlated to the duration of cross-link (scale bar: 2 μm). Image Credit: Carl Zeiss Microscopy GmbH
A longer cross-linking time results in a more pronounced merging of the fibers. A cross-linking of 120 minutes results in the mat no longer resembling a fibrous structure.
The change of the fibers leads to a change in the shape and size of the mat pores, as displayed in Figure 4. Here, individual pores are shown in different colors in 3D XRM datasets.
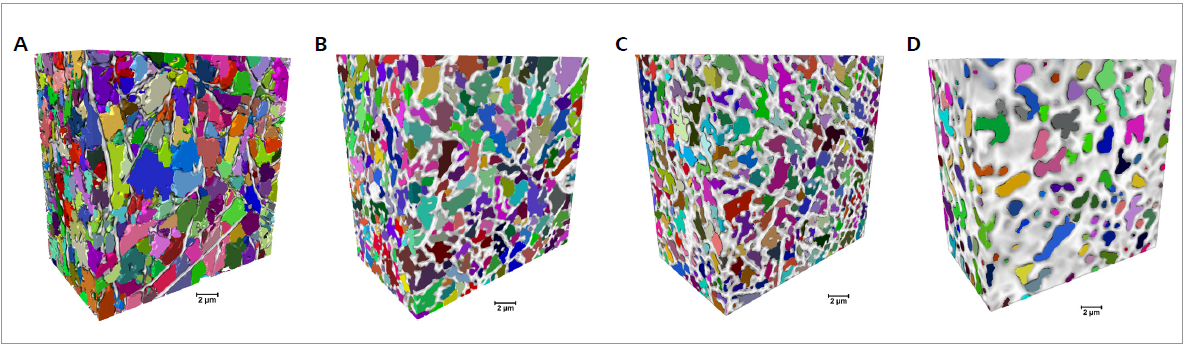
Figure 4. Pore characterization for samples cross-linked for different durations (0, 60, 90 and 120 min from A to D, respectively). Volumetric representations of the fibers (white/gray) and the samples' pore distribution, with the separated pores represented in different colors (scale bar: 2 μm). Image Credit: Carl Zeiss Microscopy GmbH
The size of the pores decreases as the cross-linking duration increases, with a more pronounced total porous volume decrease at 120 minutes of cross-linking.
Conclusion
The regeneration of damaged tissue using nanofibrous scaffolds demands the design of an extracellular matrix-like scaffold with a high surface area-to-volume ratio and high porosity for supporting homogeneous cell attachment and proliferation throughout the scaffold.
The Xradia 810 Ultra was successfully employed to characterize the morphology of electrospun gelatin fibers to understand the effect of fiber cross-linking in the gelatin mat morphology.
The Xradia Ultra 810 is a lab-based 3D X-Ray microscope that facilitates non-destructive tomography with a resolution better than 100 nm for biopolymer samples, without requiring any special sample preparation protocol.
The Xradia 810 Ultra provides phase-contrast imaging with a Zernike-type phase plate, which is unique for a lab-based microscope. This improves the quality of high-resolution imaging of low-Z materials, including biopolymers.
Phase-contrast imaging can successfully characterize the morphology of biopolymer nanofibrous scaffolds. It also provides beneficial insights for designing and fabricating novel fibrous materials.
Acknowledgments
Produced from materials originally authored by Juliana Martins de Souza e Silva, Cristine Santos de Oliveira, and Ralf B. Wehrspohn from Martin Luther University Halle-Wittenberg, Germany, Tobias Hedtke and Christian E. H. Schmelzer from the Fraunhofer Institute for Microstructure of Materials and Systems IMWS, Halle (Saale), Germany, and Fang Zhou from Carl Zeiss Microscopy GmbH, Oberkochen, Germany.
References and Further Reading
- C.S. de Oliveira, A.T. González, T. Hedtke, T. Kürbitz, A. Heilmann, C.E.H. Schmelzer, J. Martins de S. e Silva, Materials Science and Engineering: C, Volume 115, October 2020, 111045: https://doi.org/10.1016/j.msec.2020.111045
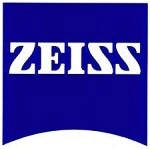
This information has been sourced, reviewed and adapted from materials provided by Carl Zeiss Microscopy GmbH.
For more information on this source, please visit Carl Zeiss Microscopy GmbH.