A major advantage of atomic force microscopes (AFMs) is their versatility in integrating various operational modes that assess different material properties and functionalities. Among the most fundamental topographical AFM modes are TappingMode and PeakForce Tapping® mode, both of which actuate the probe vertically, perpendicular to the surface. In contrast, torsional resonance (TR) modes employ horizontal actuation (parallel to the surface).
The interaction of lateral forces between the tip and the sample alters the cantilever’s resonance behavior, which can be utilized to maintain a constant distance from the surface or gather information about the sample’s properties.
Originally developed for high-resolution topographical imaging, the applications of the original torsional resonance mode (TR-Mode™) have expanded significantly. TR-Mode alone can resolve in-plane anisotropy and measure dynamic friction.
More recently, however, advancements in sensitivity have enabled the development of TR dynamic friction microscopy (TR-DFM) for high-resolution imaging of atomic lattice structures, including 2D materials. Further, by combining TR modulation with other measurement modes, additional functionalities can be introduced.
For instance, integrating tunneling atomic force microscopy (TUNA) results in TR-TUNA™, which is suitable for imaging delicate conductive samples, while combining it with magnetic force microscopy (MFM) creates TR-MFM, which can map in-plane magnetic fields.
This article serves as an introduction to and exploration of TR-based AFM modes and their diverse applications.
Introduction to Torsional Resonance Mode
A shared feature of TappingMode, PeakForce Tapping mode, and contact mode is that the principal feedback control signals are based on the cantilever probes’ static or dynamic flexural deflection.
The torsional resonance amplitude (or phase) is utilized in TR-Mode to regulate the feedback loop, keeping the associated location through lateral connection of the tip/surface. The nature of tip/surface connection of the TR-Mode enables phase calculations to determine the in-plane anisotropy of samples and dynamic friction at the nanometer scale.
In 2003, the original TR-Mode was created, patented, and introduced by Bruker as an alternative to TappingMode for high-resolution topographic imaging. TR-Mode is used for high-resolution imaging of various materials, including polymers and soft biological materials.1,2
Benefits have also been observed when imaging samples with high adhesion, where it is sometimes preferable to maintain the tip within the tip-sample adhesion zone. This approach avoids the need to increase the amplitude in vertical resonant modes, such as TappingMode, which would otherwise be necessary to retract the tip sufficiently far to escape the adhesion in every oscillation cycle.
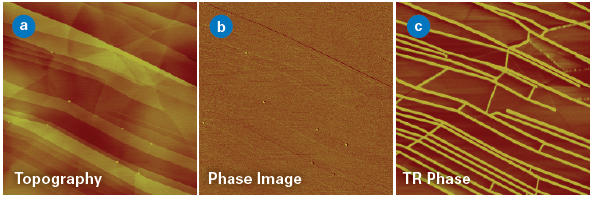
Figure 1. Freshly cleaved HOPG surface imaged with interleaved TappingMode and TR-Mode, with image sizes of 3x3 μm: (a) topography (5 nm height scale); (b) phase image obtained in TappingMode; and (c) phase image obtained in TR-Mode (both 10° scale) showing >10x higher sensitivity to water present at the steps. Image Credit: Bruker Nano Surfaces and Metrology
TR-Mode is particularly useful for samples that require highly sensitive phase detection. Generally, the Q-factor of torsional resonance is considerably higher than that of the flexural mode, which enhances sensitivity. This benefit is demonstrated in Figure 1 with a freshly cleaved, highly oriented pyrolytic graphene (HOPG) sample.
The terrace and step structures of HOPG can be clearly resolved in the tapping height image. However, the tapping phase signal does not provide additional information; the contrast merely reflects some topographic features.
In contrast, the TR-Mode phase image displays very strong contrast at some step edges. The ratio of TR-Mode phase contrast to tapping phase could be as high as a factor of 20. It is believed that the initial adsorption of water on the HOPG surface tends to concentrate around surface defects like step edges, which is evident in these phase images.
This example utilized interleave scanning, where each scan line is first collected in TappingMode, immediately followed by a TR-Mode scan of the same line.
Implementation of TR-Mode
TR-Mode requires a system capable of initiating both flexural and torsional resonances in the cantilever and seamlessly switching between controlling and measuring these two resonance modes. This is achieved using a two-element piezo control system, which is detailed in Figure 2.
Each element is coordinated with the other and automatically adjusted to minimize the coupling between torsional and flexural resonance. The NanoScope® controller utilizes lock-in amplifiers to measure the amplitude and phase of the outputs from the photodetector—vertical outputs for flexural resonances and lateral for torsional resonances.
Among Bruker AFMs, TR-Mode is available on the MultiMode®, the Dimension® Icon®, and the Dimension IconIR® series. Standard cantilevers used in TappingMode, such as the FESPA-V2—characterized by a nominal flexural free resonance frequency of 75 kHz, a tip radius of 8 nm, a length of 225 μm, and a spring constant of 2.8 N/m—are also suitable for TR-Mode.
In some cases, quantifying the TR amplitudes in nanometers may be necessary, which can be accomplished through specific calibration steps. Mullin and Hobbs have published a comprehensive quantitative comparison of two approaches to this calibration.4
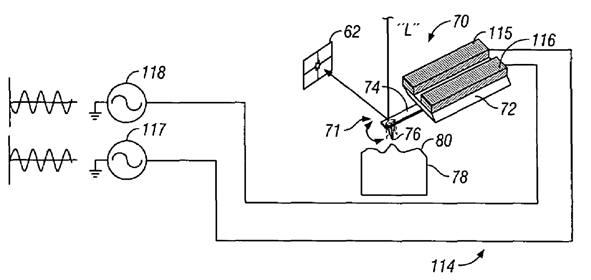
Figure 2. Schematic principle of dual piezoelectric elements to drive the probe into torsional resonance. Extracted from the Bruker Nano Inc. patent US6945099B1, “Torsional resonance mode probe-based instrument and method”. Image Credit: Bruker Nano Surfaces and Metrology
Torsional Resonance Combined with Other AFM Modes
A TR modulation can be used in various operating modes, all of which typically share the same goal of resolving in-plane (often anisotropic) material attributes.
Table 1 shows standard applications of torsional resonances. This includes the base operating mode, the corresponding feedback signal utilized for topography imaging, the properties or channels chosen, and whether LiftMode™ or interleave scanning is applied (often used as a method to deconvolute topography-related effects from the sample property measurement).
Table 1. Overview of common torsional resonance modes. Source: Bruker Nano Surfaces and Metrology
Mode |
Base mode |
Feedback Signal |
LiftMode / Interleave |
Additional Properties / Channels |
TR-Mode |
|
TR amplitude |
|
Height; TR phase |
Tapping with TR-Mode |
TappingMode |
Amplitude |
Interleave |
TR phase; TR amplitude |
TR-DFM |
Contact mode |
Deflection |
|
Dynamic friction; Contact (torsional) resonance amplitude and phase |
TR-FV |
Contact mode |
Deflection trigger |
|
Dynamic friction; Contact (torsional) resonance |
TR-EFM |
TappingMode |
Amplitude |
LiftMode |
In-plane electric field |
TR-MFM |
TappingMode |
Amplitude |
LiftMode |
In-plane magnetic field |
TR-TUNA |
|
TR amplitude |
|
Current |
Torsional Resonance Dynamic Friction Microscopy
When used with contact mode, a TR modulation results in the TR dimension and phase becoming sensitive to tribological attributes like friction. If conducted near the contact (torsional) resonance frequency with a continual standard load, the TR friction force images exhibit a much higher sensitivity than traditional contact mode–based friction calculations.
The TR friction force images are impacted less by surface topography and are usually separate from the scanning direction of the tip. This contrasts with traditional contact mode (lateral force microscopy, LFM) friction force calculations. This TR-based mode functions at a contact (torsional) resonance and is called TR dynamic friction microscopy (TR-DFM).
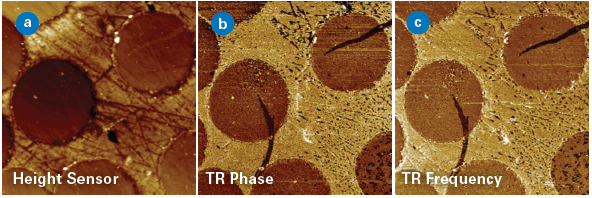
Figure 3. Cross-sectioned carbon fibers embedded in epoxy imaged (20x20 μm) with TRDFM: (a) height map from contact mode imaging and (b) TR phase image showing strong contrast related to mechanical properties of the two components. (c) PLL operation can be added to adjust the measured phase shift, creating an image of variations in the torsional contact resonance frequency. Image Credit: Bruker Nano Surfaces and Metrology
Figure 3 exhibits the outcome of TR-DFM applied to a material with cross-sectioned carbon fibers encased in epoxy.
As seen in the TR phase image (Figure 3b), TR modulation results in a strong contrast related to mechanical properties (dynamic friction and viscoelastic properties) of the two components.
A phase-locked loop (PLL) can also be applied to adjust (null) the measured phase shift, and the resulting contact (torsional) resonance frequency shifts can be extracted as an image (Figure 3c).
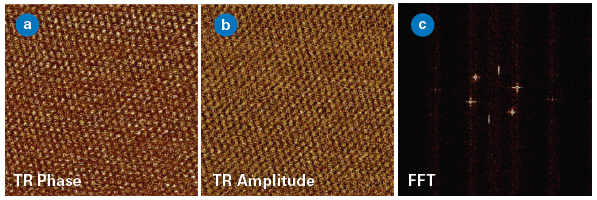
Figure 4. Atomic lattice on freshly cleaved HOPG resolved with TR-DFM (image size is 10x10 nm) using a Dimension Icon AFM and a FESPA-V2 tip with 843 kHz contact (torsional) resonance frequency: (a) TR phase image; (b) TR amplitude image, and (c) FFT image. Image Credit: Bruker Nano Surfaces and Metrology
Recently, TR-DFM has emerged as a successful approach for high-resolution imaging of atomic lattice structures, notably including 2D materials. In this case, it is important to minimize the noise and drift of the AFM and to apply small torsional resonance amplitudes. Figure 4 exemplifies this with a 10x10 nm scan of a freshly cleaved HOPG surface, where the atomic lattice structure is clearly resolved in both the TR phase and TR amplitude image.
Fast Fourier transform (FFT) analysis of the data showcases the hexagonal structure of this lattice, alongside minimal drift observed during the measurement. While similar resolution can be achieved through meticulous operation in other modes, the high sensitivity of TR-DFM yields superior signal-to-noise ratios and generally facilitates easier operation.
M. Pendharkar et al. used TR-DFM (referred to as torsional force microscopy (TFM) by the authors) to investigate a stack of atomically thin van der Waals (vdW) layers. In such layers, interlayer twist or lattice mismatch generates a moiré superlattice, the period of which is contingent upon the twist angle.
A slight change in the twist angle, even a hundredths of a degree, can alter the system’s electronic attributes substantially. Consequently, accurately determining the twist angle and mapping its spatial variation is of utmost importance.
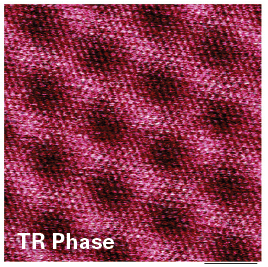
Figure 5. Moiré pattern formed by hBN layer on graphene. Both the 2.6 nm moiré pattern period as well as the underlying lattice of graphene are captured with atomic resolution in this 10x10 nm image. Data acquired with an AD-2.8-SS probe in TR-DFM at 100 nN load, 1.43 MHz contact (torsional) resonance, and 8.14 Hz line rate. Adapted from Reference 5 (DOI: 10.1073/pnas.2314083121), licensed under CC BY.
In Reference 5, TR-DFM was shown to reveal the surface and shallow subsurface structure of vdW stacks across multiple length scales:5 this includes: (i) the moirés formed between bilayers of graphene and between graphene and hexagonal boron nitride (hBN), and (ii) the atomic crystal lattices of graphene and hBN.
Figure 5 provides a high-resolution illustration, showcasing both the moiré pattern period and the underlying lattice structure of graphene. TR-DFM facilitated the precise determination of structural information, including twist angles and strain within moiré superlattices, as well as the crystallographic orientation of vdW flakes. This supports the fabrication of predictable moiré heterostructures.
Other AFM modes, such as piezoresponse force microscopy (PFM), scanning microwave impedance microscopy (sMIM), tunneling AFM (TUNA), PeakForce quantitative nanomechanics (PeakForce QNM®), etc., have also been utilized for moiré pattern imaging. However, in comparison to these modes, M. Pendharkar et al. found imaging in TR-DFM to be straightforward and highly successful.
They achieved a high success rate, detecting at least one moiré in 94 % of the 33 regions across 32 unique samples measured. Regions that did not exhibit a moiré pattern likely had relaxed to Bernal stacking. Furthermore, atomic lattices were observed at an even higher success rate.
TR-DFM was also applied to study nanoscale triboelectric effects (though the authors did not distinguish it by name and only referred to their operational mode as “TR mode”).6
Triboelectric charges produced throughout the lateral tip motion in TR-DFM were determined utilizing Kelvin probe force microscopy (KPFM). The calculated effectiveness of producing triboelectric charges was discovered to be approximately ten times greater than traditional static/contact mode.
Torsional Resonance Combined with Force Mapping
The acquisition of a force-distance curve for every image pixel across a specific area allows force volume mode to investigate a material’s morphology and calculate its mechanical attributes, including deformation, adhesion, and elastic modulus.7
In 2009, the introduction of PeakForce Tapping mode and the related PeakForce QNM modes provided a powerful alternative method for the measurement of mechanical properties.
These force mapping methods (force volume and PeakForce QNM) are now widely adapted to simultaneously obtain information on sample morphology and mechanical properties at the surface with extremely high lateral resolution.
Force mapping-based methods can also be combined with TR-DFM: during the contact time between the tip and sample in each force-distance cycle, a TR oscillation can be applied, and the corresponding TR phase and amplitude can be collected in the same way as in TR-DFM.
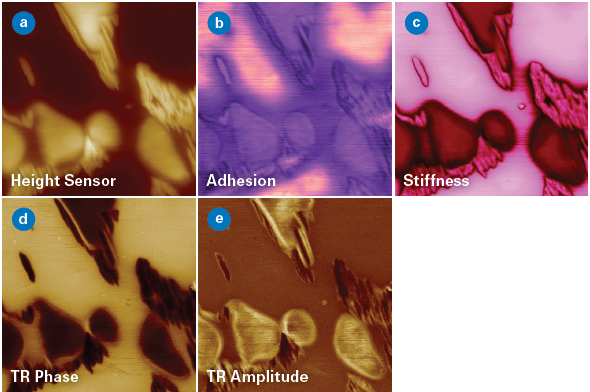
Figure 6. TR-DFM performed during force volume imaging on a PDES polymer sample, with image sizes of 10x10 μm. (a) Height was measured during force volume imaging. (b) Adhesion and (c) stiffness were extracted from the approach and retract segments in force volume mode, while the torsional resonance signals (d) TR phase and (e) TR amplitude were extracted during the contact time and relate to the shear-force and frictional properties of the sample, respectively. Image Credit: Bruker Nano Surfaces and Metrology
Figure 6 illustrates a combined force volume and TR-DFM on a PDES sample on Si substrate.
TR phase and TR amplitude information were collected at the exact locations as the regular force volume image channels (height, adhesion, stiffness, modulus), enabling correlated imaging of various mechanical properties. Each image channel exhibits unique information arising from different contrast mechanisms, highlighting the complementarity of this combined method.
PeakForce Tapping and TR-Mode can also be combined, leveraging the advantages of PeakForce QNM over force volume imaging: (i) faster operation speeds, with peak force cycles of 2 kHz, (ii) reduced operating forces, and (iii) enhanced force control facilitated by the built-in peak force feedback mechanism.
In Reference 8, the authors introduce a data processing and multidimensional mechanical information extraction algorithm based on the composite mode of PeakForce Tapping and TR-Mode.
Through the separation of transverse and longitudinal mechanical information, they achieved both quantitative measurements of longitudinal mechanical properties from PeakForce Tapping and qualitative analysis of transverse mechanical properties from TR-Mode.
Using this approach, they developed an optimized algorithm for synchronizing AFM images and proposed a method to accurately identify the contact area between the tip and sample for each peak force cycle, thus enabling the collection of more precise information regarding morphology and mechanical properties.
Torsional Resonance Combined with Magnetic Force and Electric Force Microscopies
The ability to switch between flexural and torsional resonance within scan lines can also be employed in magnetic force microscopy (MFM) and torsional resonance MFM (TR-MFM). While conventional MFM based on Tapping Mode is sensitive to the vertical component of magnetic fields, TR-MFM selectively detects the field with a force gradient parallel to the tip.
By acquiring both force gradient components, a new level of information is obtained, leading to a better understanding of the force and its gradient source. The in-plane force gradient components can be measured with the same resolution and signal-to-noise ratio as flexural modes at the same location.9,10
Studies assessing TR-MFM for imaging magnetic structures have demonstrated two advantages over conventional MFM: (i) the ability to acquire magnetic contrast free from topographic influence and (ii) a 15 % enhancement in lateral resolution.11
This improvement in lateral resolution is directly linked to maintaining a constant tip-sample separation at extremely small values, in contrast to conventional MFM, where tip-sample separation varies over larger ranges. This resolution enhancement applies to any magnetic tip; hence, combining TR-MFM with ultra-sharp magnetic probes can further optimize magnetic lateral resolution.
Additionally, since electric force microscopy (EFM) operates on the same principles as MFM, TR-Mode can also be utilized for TR-EFM and TR-KPFM applications.12
Torsional Resonance Combined with Tunneling Atomic Force Microscopy
Several nanoelectrical atomic force microscopy modes rely on contact mode, as they necessitate tip-sample contact to measure the electrical property of interest, such as conductivity.
However, traditional contact mode techniques like conductive AFM (C-AFM) and tunneling AFM (TUNA) are unsuitable for soft and fragile materials, such as certain organic materials or nanowires. These materials may exhibit measurement artifacts like plowing or material piling up due to the high lateral forces inherent in contact mode operation.
To address this challenge, intermittent-contact electrical methods like Bruker's PeakForce TUNA™ or force volume (utilized in DataCube methods) have emerged as key solutions.
These methods have gained popularity among researchers due to their primary advantages: (i) absence of lateral forces, enabling imaging of soft and fragile samples, achieving higher spatial resolution, and prolonging tip lifetime; (ii) capability to facilitate correlated mechanical and electrical property characterization.
Using TUNA with a TR modulation (TR-TUNA) provides an alternative method for overcoming the restrictions of contact mode–based electrical calculations as the TR amplitude feedback keeps the tip material distance steady with a minimal value. This distance is low enough to allow calculations of material conductivity.
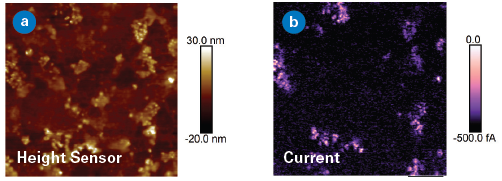
Figure 7. TR-TUNA (a) height and (b) current 5x5 μm images acquired on a carbon sticky tape at -0.5 V sample bias. The carbon nanoparticles which form a percolation path to the back substrate show up in the TR-TUNA current image. Image Credit: Bruker Nano Surfaces and Metrology
TR-TUNA was created and patented by Bruker and is utilized for mapping conductivity in various materials with high spatial resolution: carbon tape (Figure 7), nanofibrillar-polyaniline/carbon nanotube composites,13 a conducting polymer blend of poly(3,4-ethylenedioxythiophene) poly(styrene sulfonate),14 vertical nanorods and pillar array structures,15 nanoparticles,16 and thin dielectric and organic semiconductor films17.
Summary
TR-Mode was initially developed only for topographical imaging, but its value is now much broader. TR-based modes can resolve in-plane anisotropy and measure dynamic friction. TR-DFM introduces the ability to image atomic lattice structures, even for 2D materials. Combining the TR modulation with other modes results in unique capabilities like imaging delicate conductive samples and mapping in-plane magnetic fields.
Acknowledgments
Produced from materials originally authored by Bede Pittenger, Shuiqing Hu, Chanmin Su, Peter De Wolf, all from Bruker.
References and Further Reading
- N. Mullin, C. Vasilev, J.D. Tucker, et al. 2009. “‘Torsional Tapping’ Atomic Force Microscopy Using T-Shaped Cantilevers.” Appl. Phys. Lett. 94 (17): 173109. DOI: 10.1063/1.3126047
- R.C. Savage, N. Mullin, and J.K. Hobbs. 2015. “Molecular Conformation at the Crystal–Amorphous Interface in Polyethylene.” Macromolecules 48 (17): 6160–65. DOI: 10.1021/ma5025736
- L. Huang and C. Su. 2004. “A Torsional Resonance Mode AFM for In-Plane Tip Surface Interactions.” Ultramicroscopy 100 (3–4): 277–85. DOI: 10.1016/j. ultramic.2003.11.010
- N. Mullin and J. Hobbs. 2014. “A noncontact, thermal noise-based method for the calibration of lateral deflection sensitivity in atomic force microscopy,” Rev. Sci. Instrum. 85, 113703, DOI: 10.1063/1.4901221
- M. Pendharkar, S.J. Tran, G. Zaborski Jr, et al. 2024. “Torsional force microscopy of van der Waals moirés and atomic lattices.” PNAS 121(10): e2314083121. DOI: 10.1073/pnas.2314083121
- Cai, Wei, and Nan Yao. 2016. “Dynamic Nano-Triboelectrification Using Torsional Resonance Mode Atomic Force Microscopy.” Sci. Rep. 6 (1): 27874. DOI: 10.1038/srep27874
- M. Radmacher, J.P. Cleveland, M. Fritz, et al. 1994. “Mapping interaction forces with the atomic force microscope.” Biophys. J. 66: 2159–2165. DOI: 10.1016/s0006-3495(94)81011-2
- C. Hao, S. Wang, S. Yuan, et al. 2022. “Extraction Algorithm for Longitudinal and Transverse Mechanical Information of AFM.” Nanotechnol. Precis. Eng. 5 (2): 023004. DOI: 10.1063/10.0010252
- C. Su, L. Huang, P. Neilson, et al. 2003. “In-Situ Measurement of in-Plane and out-of-Plane Force Gradient with a Torsional Resonance Mode AFM.” AIP Conf. Proc. 696: 349. DOI:1063/1.1639717 10
- T. Kasai, B. Bhushan, L. Huang, et al. 2004. “Topography and Phase Imaging Using the Torsional Resonance Mode.” Nanotechnology 15 (7): 731–42. DOI: 10.1088/0957-4484/15/7/004
- A. Kaidatzis and J.M. García-Martín. 2013. “Torsional Resonance Mode Magnetic Force Microscopy: Enabling Higher Lateral Resolution Magnetic Imaging without Topography-Related Effects.” Nanotechnology 24 (16): 165704. DOI: 10.1088/0957- 4484/24/16/165704
- H. Zhang, H. Gao, J. Geng, et al. 2022. “Torsional Harmonic Kelvin Probe Force Microscopy for High-Sensitivity Mapping of Surface Potential.” IEEE Trans. Ind. Electron. 69 (2): 1654–62. DOI: 10.1109/TIE.2021.3057040
- W.K. Maser, P. Jiménez, N.O. Payne, et al. 2009. “Nanofibrillar-Polyaniline/ Carbon Nanotube Composites: Aqueous Dispersions and Films.” J. Nanosci. Nanotechnol. 9 (10): 6157–63. DOI: 10.1166/jnn.2009.1578
- S.A.L. Weber and R. Berger. 2013. “Electrical Tip-Sample Contact in Scanning Conductive Torsion Mode.” Appl. Phys. Lett. 102 (16): 163105. DOI: 10.1063/1.4802725
- S.A.L Weber, N. Haberkorn, P. Theato, et al. 2010. “Mapping of Local Conductivity Variations on Fragile Nanopillar Arrays by Scanning Conductive Torsion Mode Microscopy.” Nano Lett. 10 (4): 1194–97. DOI: 10.1021/nl9035274
- C. Prastani, A. Vetushka, A. Fejfar, et al. 2012. “Conductivity Mapping of Nanoparticles by Torsional Resonance Tunneling Atomic Force Microscopy.” Appl. Phys. Lett. 101 (8): 083107. DOI: 10.1063/1.4744601
- A. Hofer, R. Biberger, G. Benstetter, et al. 2013. “Scanning Probe Microscopy Based Electrical Characterization of Thin Dielectric and Organic Semiconductor Films.” Microelectron. Reliab. 53 (9–11): 1430–33. DOI: 10.1016/j. microrel.2013.07.086
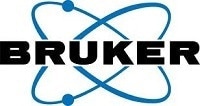
This information has been sourced, reviewed and adapted from materials provided by Bruker Nano Surfaces and Metrology
For more information on this source, please visit Bruker Nano Surfaces and Metrology