Sponsored by MerckFeb 1 2024Reviewed by Emily Magee
Silver nanomaterials possess distinctive physical, chemical, and optical characteristics that are used for a diverse array of applications.
The renewed interest in silver as a comprehensive antimicrobial agent has resulted in the creation of numerous products incorporating silver nanoparticles to hinder bacterial growth on various surfaces and in clothing items.
The optical features of silver nanoparticles are intriguing due to their robust coupling with specific wavelengths of incident light.
This attribute allows for an adjustable optical response, enabling the development of exceptionally bright reporter molecules, highly effective thermal absorbers, and nanoscale "antennas" that enhance the strength of the local electromagnetic field, facilitating the detection of alterations in the nanoparticle environment.
This article summarizes the importance of precisely engineering silver nanoparticle size and shape for various applications.
Morphology and Surface Functionalization
Reaction conditions during silver nanomaterial fabrication can be tuned to produce colloidal silver nanoparticles with a variety of morphologies, including monodisperse nanospheres, triangular prisms or nanoplates, cubes, wires and nanorods (Figure 1).
For many applications, careful selection of surface chemistry, morphology, and optical properties associated with each nanoparticle variant is essential to achieve the desired functionality in the target environment.
The surface chemistry of these particles, encompassing binding strength, functional groups, and the size of the capping agents coating the particles, can be varied to exert an additional level of control over particle behavior.
In aqueous media, numerous nanoparticles gain electrostatic stabilization through the introduction of charged species at the particle surface. The surface charge of silver nanoparticles can be regulated by coating them with citrate ions, providing a robust negative charge.
Alternatively, substituting citrate ions with branched polyethylenimine (BPEI) results in an amine-dense surface with a highly positive charge.
Various capping agents offer additional functionality; polyethylene glycol (PEG)-coated nanoparticles exhibit stability in high-salt-concentration solutions, while lipoic acid-coated particles with carboxyl groups can be employed for bioconjugation.
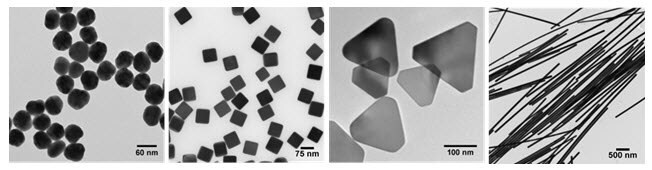
Figure 1. Transmission electron micrographs demonstrating the diversity of size and morphology possible by control over the reaction chemistry and kinetics during the solution-phase synthesis of silver nanomaterials: (left to right) uniform 50 nm diameter spheres, 75 nm cubes, 120 nm triangular nanoplates, and silver nanowires. Image Credit: Merck
Optical Properties
Silver and other noble metal nanoparticles exhibit strong interaction with light because the conduction electrons on the metal surface undergo a collective oscillation when they are excited by light at specific wavelengths.
This oscillation, known as a surface plasmon resonance (SPR), causes the absorption and scattering intensities of silver nanoparticles to be much higher than identically sized non-plasmonic nanoparticles.
Silver nanoparticle absorption and scattering properties can be tuned by controlling the particle size, shape, and the local refractive index near the particle surface.
Figure 2 illustrates the extinction spectra of silver nanospheres, nanoplates of different sizes, and the appearance of dilute nanoparticle dispersions.
Smaller nanospheres primarily absorb light with plasmon resonance peaks around 400 nm, while larger spheres exhibit increased scattering with peaks broadening and shifting towards longer wavelengths.
Silver nanoplates, due to their anisotropic shape, possess exceptionally large absorbing and scattering cross-sections across the visible and near-IR regions, allowing precise tuning of plasmon resonance peaks at specific wavelengths by controlling plate diameter and thickness (Figure 2).
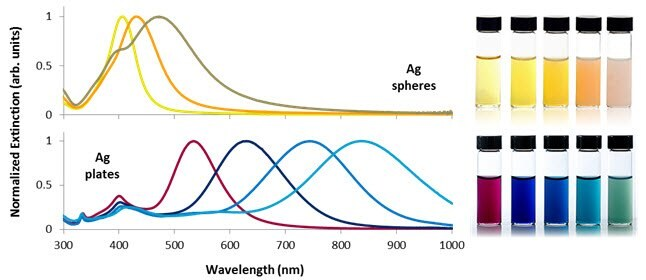
Figure 2. Selected optical extinction spectra and solution appearance of silver nanospheres between 10 and 100 nm in diameter (top) and silver nanoplates between 50 and 150 nm in diameter (bottom). Control over nanoparticle shape and size allows the plasmon resonance to be tuned across the visible and near-infrared portions of the spectrum. Image Credit: Merck
Anti-Microbial Properties
Silver ions are an effective antimicrobial due to their interaction with thiol groups of vital bacterial enzymes and proteins affecting cellular respiration, resulting in cell death.
The distinctive property of silver to exhibit high toxicity towards bacteria while maintaining minimal toxicity to humans has facilitated its integration into a diverse array of products, including wound dressings, packaging materials, and anti-fouling surface coatings.
A fundamental mechanism of silver nanoparticle antimicrobial activity lies in their role as a high surface area source for silver ions. In aqueous environments, the particles oxidize in the presence of oxygen and protons, releasing Ag+ ions as the particle surface dissolves.
The sustained release and maintenance of an effective concentration of silver ions in various solutions underpins silver nanoparticles' long-term antimicrobial efficacy.
The release rate of silver ions depends on factors such as size, shape, capping agent, aggregation state, and the environment. Smaller or anisotropic silver nanoparticles typically exhibit faster ion release rates due to the high surface energy of curved or strained nanoparticle surfaces.
The nanoparticle environment, including elevated temperatures and the presence of chlorine, thiols, and oxygen, also strongly influences ion release.
Tagging and Targeting for Bioimaging
Silver nanoparticles exhibit remarkable light absorption and scattering efficiency, finding extensive use in tagging and imaging applications. The high scattering cross-section enables the visualization of individual silver nanoparticles using dark field microscopy (Figure 3) or hyperspectral imaging systems.
By linking biomolecules like antibodies or peptides to the nanoparticle surface, silver nanoparticles can be directed toward specific cells or cellular components.
Attachment of targeting molecules to the surface can be done via physisorption onto the nanoparticle surface or through covalent coupling, such as the use of ethyl(dimethylaminopropyl) carbodiimide (EDC) to link free amines on an antibody to carboxyl groups present on lipoic acid-capped nanoparticles.
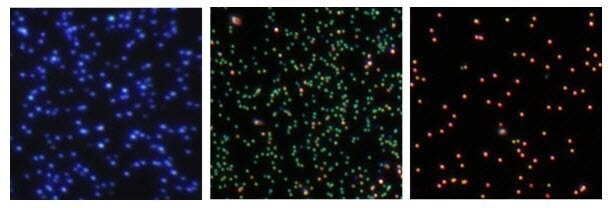
Figure 3. Dark field microscopy images of (left to right) 60 nm diameter silver nanospheres, 75 nm diameter silver nanocubes, and 100 nm diameter silver nanocubes, illustrating the ability to tune the scattering color of silver nanoparticle labels based on size and shape. Image Credit: Merck
In biological applications, the use of silver nanoparticles is also based on leveraging the enhanced electromagnetic field at and near their surface. Acting as nanoscale antennas, particularly at the plasmon resonance wavelength, these silver nanoparticles amplify the intensity of the local electromagnetic field.
One spectroscopic technique benefiting from this enhancement is Raman spectroscopy, which identifies molecules by their distinct vibrational modes.
While intrinsic Raman scattering from molecules is feeble and requires extended measurement times, Surface Enhanced Raman Scattering (SERS) from molecules close to plasmonic silver nanoparticles offers significantly intensified Raman signals.
The SERS effect elevates the Raman scattering of attached molecules by up to 14 orders of magnitude, enabling the detection of even single molecules.
This intensified field effect can also boost fluorescence by positioning a fluorophore near a silver nanoparticle, leading to Surface Enhanced Fluorescence (SEF). SEF potentially augments fluorophore emission intensity by substantial orders of magnitude.
This enhancement arises from two effects:
- The concentration of incoming light due to the large absorption and scattering cross-sections of the plasmonic particle.
- A reduction in the fluorescence lifetime of the fluorophore, enabling the excited state to revert to the ground state at a higher frequency.
Effects like these are heavily reliant on how well the optical properties of the fluorophore and nanoparticle align, along with where the fluorophore sits in relation to the particle.
Figure 4 outlines various attachment approaches crucial for achieving optimal SERS and SEF effects with a dye molecule.
Attaching a dye molecule to a metal nanoparticle typically results in quenching of the emission due to energy transfer between the excited state of the fluorophore and the electronic states of the metal. However, the Raman spectrum of the molecule is strongly enhanced due to the high electromagnetic field at the surface of the particle (Figure 4, A).
By positioning the fluorophore at a slight distance from the particle surface, fluorescence quenching is prevented while still maintaining the high local electromagnetic field. This strategic spacing leads to a significant enhancement in the photoemission from the molecule.
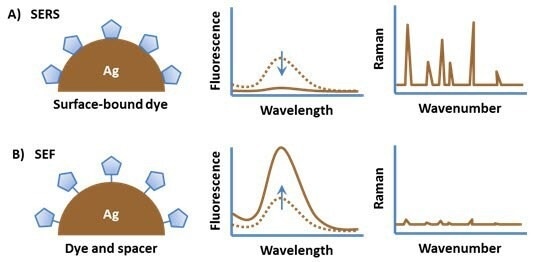
Figure 4. (A) An organic fluorophore attached directly to a metal substrate typically has quenched fluorescence but a strong surface enhanced Raman spectrum. (B) Spacing the fluorophore off of the metal surface results in surface enhanced fluorescence. Image Credit: Merck
Nanomedicine and Nanotoxicology
The use of silver nanoparticles in in-vitro and in-vivo applications is rapidly increasing. In addition to silver nanoparticle-based labeling and nanotags, other applications for silver nanoparticles include use as thermal sources for hyperthermia and thermally modulated release from particle surface coatings.
Silver nanoparticles can also be incorporated into core/shell constructs, in which an amorphous silica shell is grown uniformly onto silver nanoparticle seeds. These shells can accommodate various functional groups, enabling the integration of fluorophores, drug molecules, or other high-molecular-weight organic compounds for labeling or drug delivery purposes.
Future biomedical applications of silver nanoparticles will necessitate a comprehensive understanding of their interaction with biological systems.
Designing particles for in-vivo applications poses a significant challenge, demanding prolonged circulation times and minimal toxicity. Optimizing nanoparticle performance within in-vivo systems through experiments is quite complex due to the intricacies of both the nanoparticles and their environment.
The biological fate and movement of nanoparticles hinge not only on their primary attributes (e.g., core chemistry, size, shape, crystallinity, surface, and aggregation state) but also on secondary attributes influenced by their interaction with target biological systems (e.g., protein corona, dissolution rate, biodistribution).
Summary
The unique optical and broad-based antimicrobial properties of silver nanoparticles have led to a rapid rise in the incorporation of silver nanoparticles in biological applications.
The precise manipulation of their size, shape, and surface attributes presents a robust toolbox not only for crafting functional biological materials but also for comprehending the fundamental mechanisms governing nanoparticle transport and interaction within biological systems.
This understanding, coupled with the development of intricate multifunctional silver nanocomposites, is set to usher in the next era of probes, devices, and therapeutics based on silver nanoparticles.
Merck’s Materials Science portfolio includes an assortment of meticulously characterized silver nanomaterials—ranging from nanoparticles and nanospheres to nanoplates—each tailored with specific surface functionalities ideal for diverse biomedical applications.
References
- Rai M, Yadav A, Gade A. 2009. Silver nanoparticles as a new generation of antimicrobials. Biotechnology Advances. 27(1):76-83. https://doi.org/10.1016/j.biotechadv.2008.09.002
- Marambio-Jones C, Hoek EMV. 2010. A review of the antibacterial effects of silver nanomaterials and potential implications for human health and the environment. J Nanopart Res. 12(5):1531-1551. https://doi.org/10.1007/s11051-010-9900-y
- Sondi I, Salopek-Sondi B. 2004. Silver nanoparticles as antimicrobial agent: a case study on E. coli as a model for Gram-negative bacteria. Journal of Colloid and Interface Science. 275(1):177-182. https://doi.org/10.1016/j.jcis.2004.02.012
- Kim JS, Kuk E, Yu KN, Kim J, Park SJ, Lee HJ, Kim SH, Park YK, Park YH, Hwang C, et al. 2007. Antimicrobial effects of silver nanoparticles. Nanomedicine: Nanotechnology, Biology and Medicine. 3(1):95-101. https://doi.org/10.1016/j.nano.2006.12.001
- Carlson C, Hussain SM, Schrand AM, K. Braydich-Stolle L, Hess KL, Jones RL, Schlager JJ. 2008. Unique Cellular Interaction of Silver Nanoparticles: Size-Dependent Generation of Reactive Oxygen Species. J. Phys. Chem. B. 112(43):13608-13619. https://doi.org/10.1021/jp712087m
- Moskovits M. 1978. Surface roughness and the enhanced intensity of Raman scattering by molecules adsorbed on metals. The Journal of Chemical Physics. 69(9):4159-4161. https://doi.org/10.1063/1.437095
- Kneipp K, Wang Y, Kneipp H, Perelman LT, Itzkan I, Dasari RR, Feld MS. Single Molecule Detection Using Surface-Enhanced Raman Scattering (SERS). Phys. Rev. Lett.. 78(9):1667-1670. https://doi.org/10.1103/physrevlett.78.1667
- Geddes CD, Lakowicz JR. 2002. 12(2):121-129. https://doi.org/10.1023/a:1016875709579
- Powers KW, Palazuelos M, Moudgil BM, Roberts SM. 2007. Characterization of the size, shape, and state of dispersion of nanoparticles for toxicological studies. Nanotoxicology. 1(1):42-51. https://doi.org/10.1080/17435390701314902
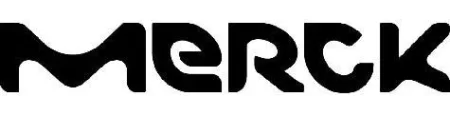
This information has been sourced, reviewed and adapted from materials provided by Merck.
For more information on this source, please visit Merck.