Asylum Research has two AFM families, the Cypher TM and MFP-3DTM which can evaluate 3D textural and roughness characteristics quantitatively, at unparalleled resolution and with the ability to assess functions at nanoscale.
Today, thin films and coatings are vital parts of all kinds of applications, from photovoltaics to food containers. They are therefore manufactured from a huge range of material classes to suit these different needs, and their production includes many and varied processes, such as physical or chemical vapor deposition, atomic layer deposition and sol-gel transitions.1 However, every new film must have both the surface structure and the physical properties characterized, whether it is for the purpose of manufacturing a commercially available product or for a project in materials science, as shown in Figures 1 and 2 respectively.
Films have intrinsic dimensions, such as the thickness of the film, the size of the grains and of the domain, which means measurements have to be taken frequently on micrometer to subnanometer scales. Many factors fit AFMs for this role. One is the much greater spatial resolution available with this method, compared to either optical or stylus-based techniques.4
Another is its ability to work with non-optically reflective or electrically conducting samples, which means a virtually unrestricted range of films can be examined. Again, the AFM data complements that obtained by electron microscopes, such as more reliable 3D profiles of a surface. It also allows more latitude with respect to the operating environment so that the work can be executed at ambient or non-ambient temperatures and atmospheres.
This article shows how Asylum Research AFMs are used to accomplish thin film characterization over numerous types of applications. Modern AFMs allow the fastest ever measurement of surface roughness, with precision and ease. Many more analysis tools and automated protocols are integrated into the AFM for more productivity and easier use. Advanced designs have also allowed more AFM modes to evolve for the assessment of functionality at nanoscale, such as magnetic, electrical and mechanical properties.
.jpg)
Figure 1. Oxygen plasma treatment of polyethylene terephthalate (PET) films. PET fibers coated with a conducting polymer such as polypyrrole could be used in “smart” electronic textiles. However, achieving good coating-to-fiber adhesion remains a key challenge. Images of PET films exposed to oxygen plasma show that RMS surface roughness increased with exposure time. Films processed longer than 60 s displayed surface etching and uniform nanoscale features. The graph reveals a linear dependence of roughness on treatment time after ~30 s. Combined with data on surface chemistry, the results can be used to optimize treatment parameters for improved coating adhesion and conductivity. Scan size 1 μm; height scale 35 nm. Imaged with the Cypher S AFM. Adapted from Ref. 2.
.jpg)
Figure 2. Strain effects in ferroelectric NaNbO3 (NNO) films grown on TbScO3 (TSO) substrates with metal organic chemical vapour deposition (MOCVD). Growth of epitaxial NNO on TSO results in significant anisotropic misfit strain. Understanding relations between strain, crystal structure, and ferroelectric response will enable finetuning of film properties. The lateral piezoresponse force microscopy (PFM) image on a film with thickness d=11 nm reveals a strong in-plane piezoresponse with highly ordered domains (vertical stripes). For a thicker film (d=21 nm), distortions in the alignment appear. For an even thicker film (d=66 nm), 90o domains (horizontally striped regions) are observed, indicating a 1D to 2D domain pattern transformation. The graph shows values for the lateral piezoelectric domain width D obtained by PFM and x-ray diffraction (XRD).The dependence of D on d changes from approximately constant to the predicted D∝d0.5 (dotted line) at d≈20 nm, where the 1D to 2D transformation occurs. Acquired on the MFP-3D AFM.Adapted from Ref. 3.
Imaging Topography
The way a film behaves is closely correlated with its surface structure, like its roughness and texture. One instance is the influence that grain size bears to electromigration within a copper interconnect. Another is the way that the microstructure of carbon coatings, similar to diamond on cutting tools, affects the friction produced. Another property of the surface, namely, roughness, underlies the wettable characteristics of various biocompatible coatings designed to be applied to medical implants. Similarly, if the roughness is controlled by nanopattern production on the surface, LED efficiency is boosted.
The ability to evaluate the roughness of a surface during the product development cycle is useful in measuring process control efficiency as well as in uncovering the way the structure affects the property of the material. Measurements are also of use when it comes to elucidating the effects of post deposition treatments, such as heat, plasma or polishing treatment, and to reveal the modes of product failure.
AFM can be used to obtain 3D images of the topography or height of the surface features, allowing better understanding of the nanostructure of the film: its roughness, defects, amorphous phase and crystalline structure, as well as nucleation and the pattern of growth. The tapping mode is ideal for topographic analysis. In this mode, the cantilever of the AFM is oscillated to the borderline of resonance frequency.
The probe tip comes into contact with the surface of the sample only for a part of the cycle of oscillation. This reduces the chances of damage to the sample as well as to the probe tip because the forces exerted both laterally and vertically between the tip and the sample surface are so much lighter.
This mode has a high spatial resolution because of the extreme sharpness of the tip, and the resulting smallness of the area in contact with the sample. This allows structures to be resolved to atomic and crystal level, as Figure 3 and 4 show.
AFM topography leads to very precise measurements if both Z (vertical) and XY (lateral) directions have good spatial resolution. Vertical resolution is determined mostly by instrument noise. This in turn depends upon mechanical vibrations and any random fluctuations in the electrical current, which can cause the height signal to vary. Any vertical features of smaller dimension than this variation in height signal cannot be resolved with clarity.
Another factor affecting accuracy is Z-piezolinearity that is noted as the sample becomes rougher. The lateral resolution depends mainly on how big the AFM cantilever tip is, which determines its impact on the area of contact between the tip and the sample. A new probe tip is generally less than 10 nm, which typically allows a contact radius of below 1 nm between tip and sample in the tapping mode.
The issue is the slow wearing down of the tip which impacts the lateral resolution as well as the precision or repeatability of the measurement. This blunting of the tip can be prevented if there is a responsive mechanical Z-axis on the AFM. This rapidly adjusts the base of the cantilever as it senses the variation in surface height, thus ensuring that the pressure on the tip is always as intended and so protecting it from unnecessary wear.
.jpg)
Figure 3. Topography imaging of atomically flat strontium vanadate (SVO). The epitaxial film was grown by pulsed electron-beam deposition and was ~6.5 nm thick. SVO has potential applications in electronic switching and sensing, and recent advances in synthesis techniques enable growth of low-dimensional, ultrathin films with improved functionality. The image was acquired with the Cypher S AFM and demonstrates its superb Z resolution. Height scale 0.9 nm. The section across the line in the image shows the atomically flat nature of the film, with step-terrace structures high corresponding to the lattice spacing of one unit cell. Adapted from Ref. 5.
Asylum Arms for Imaging the Topography
- Both Cypher and MFP-3D AFMs have the most advanced position sensors which have extremely low noise, down to 35 pm in the Z axis with the Infinity instrument, and 60 pm in both X and Y with the Cypher family. They incorporate closed-loop scanners to ensure that scanning movements are performed with accuracy and repeatability.
- The use of cantilevers smaller than 10 microns allows lines to be scanned at up to 40 Hz on a routine basis on the Cypher instruments, to complete a full 256x156 pixel image in a mere 5-10 seconds.
- In most settings, Cypher AFMs can work without vibration isolation fittings, even when the sample being imaged is atomically flat. Their design integrates maximum stability to mechanical forces and they are fitted into an acoustic enclosure already. As a result they hardly sense normal noise in the surroundings, decreasing the thermal drift to a level ten times lower compared to earlier AFMs.
- The use of GetStartedTM allows imaging parameters to be optimized automatically, to make the tapping mode extremely convenient. Both the MFP-Infinity and Cypher AFMs use a predictive algorithm to define the parameters before the first tip-sample contact, which allows the data to reach high quality from the very start.
- The use of the automated laser alignment tool SpotOnTM in Cypher AFMs brings down the time required for setting up the instrument. Just one click on the position required is sufficient to align the laser spot on the cantilever arm, as well as to make sure the photodetector is centered.
|
Another problem with normal topography measurements is the occurrence of image drift, which reduces the accuracy and repeatability of the image. This primarily follows thermal variations such as room temperature differences. Such variations can produce expansion and contraction of the components of the AFM leading to a shift in the relative positions between the sample and cantilever. To prevent this, the design must be adapted with care or the temperature of the AFM must be diligently regulated.
Besides the better spatial resolution, other technical improvements have also been made to Asylum AFMs. Some include the tools for automated setup, and multiple programs for data analysis. The speed with which images are acquired has also gone up dramatically.
The increase in scanning speed leads to a higher throughput, such as when a number of sample regions are imaged to evaluate the uniformity of a film. Such high scans can be achieved with the Cypher because of the higher resonant frequencies possible with smaller cantilevers, which are also associated with less noise and improved resolution, so that very low forces in the range of piconewtons are better resolved and controlled. Such benefits allow Cypher AFMs to routinely image single-point atomic defects or the double helix of the DNA molecule.
.jpg)
Figure 4. Temperature-dependent structural transformations in epitaxial BiFeO3 (BFO) films. Highly strained BFO is a multiferroic material that exhibits complex structural changes near room temperature. Understanding how these changes affect the electromechanical response will hasten applications in sensors, actuators, and electronic memory. (left) Topography images for a 110 nm thick film reveal regions of atomically flat terraces (blue) and mixed-phase regions (red) that evolve with temperature. Height scale 8 nm. Acquired with the PolyHeater stage on the MFP-3D AFM. (right) Structural parameters as a function of sample temperature and film thickness. (top) The percentage of mixed phase was calculated by masking the topography images to determine the areal ratio of mixed-phase regions to the entire sample area. (bottom) RMS roughness is an indicator of the volume fraction of mixed-phase regions in the films. Adapted from Ref. 6.
Using Asylum AFMs to Analyze Surface Roughness
- The roughness measures evaluated with the Asylum AFMs include the following:
.jpg)
- The Asylum AFM can be used to calculate these along with other surface parameters because of the integrated software. All the calculations can be modified or validated by the user, and if desired the user can add in more parameters or algorithms.
- The Asylum AFM software also incorporates a number of topography analytical tools. These include masks which can either include or exclude any region from the analysis. The use of thresholding protocols help to bring up specific regions of study or masks. Height value distributions may be shown through histograms and sections. Spatial Fourier transforms for power spectral density, and pore or grain analysis, are also included.
|
Roughness Analysis
Parameters used to analyze roughness bring together the whole mass of 3D information derived from topographical imaging. One example is in imaging roughness. The three main parameters used include amplitude, spacing and hybrid. Amplitude describes the surface on the basis of the variations in the Z axis, but spacing denotes variations in XY. Hybrid parameters are designed to characterize X, Y and Z variations.
The most common parameters used are the amplitude parameters, such as the average deviation Ra or Adev, the root mean square RRMS, and the standard deviation Rq or Sdev which measure length. Thus the higher the value, the greater the height variation. However, these parameters can show the same values even with dramatically different sample textures, because they do not differentiate peaks from valleys or show the spacing of features.
RRMS and Sdev can reflect non-uniform highs and lows with more sensitivity than Adev. RRMS and Sdev show height variation dispersion, but the latter is generally smaller in value as it fails to include the average height. A systematic examination of topographical images is shown in Figure 4, performed in order to study multiferroic films.
Kurtosis and skewness are terms used to describe roughness parameters without dimensions, to describe how height variations are distributed in space. If the distribution is normal, the skewness Rsk and kurtosis Rku are zero in value. Skewness is a property which shows how symmetrically the height variation is distributed about the mean line. Surfaces with high peaks and spikes have Rsk values over zero, but when it is less than zero it denotes the presence of deep valleys or pits.
Kurtosis shows how uniform height variations are, such that Rku is over zero for surfaces with multiple peaks and valleys, but less than zero if the surface is relatively smooth with fewer spikes and pits.
When an AFM is used to measure surface roughness, variables such as the tip radius and scan size influence the result, as with any experimental instrument.4 It cannot sense any feature whose dimension is less than that of the tip diameter, especially pits and valleys. For this reason, parametric measures like Adev show a negative tendency as the tip size goes up. Also, any feature bigger than the scan size cannot be detected either.
An image which has a larger scan size will show a tendency to increasing roughness values as the instrument detects features which have both long and short spatial wavelengths. Thus for any valid comparison of roughness values, the tip and imaging conditions should be maintained at similar conditions to the greatest extent possible.
Examining Solvent and Heat Effects
When the device is being integrated into the system or the product is being used, it is possible to expose the film to non-ambient conditions like liquid solvents, vapor solvents, or temperature variations. If these variations are simulated during the AFM measurement, the results gain more meaning and the analysis of long-term dependability and sturdiness can be carried out.
Research into fundamental materials also gains from understanding how measurements of the topography, functionality and mechanical characteristics are altered, with variations in solvent concentrations or temperature.
Asylum AFMs are built to operate in controlled surroundings, achieving accurately regulated and very stable temperatures and heating rates. Figure 4 shows one example where multiferroic films are being measured for topography at up to 300 oC, while Figure 5 follows changes in the morphology of a block copolymer film as it is exposed to toluene vapor. To employ AFM in liquid or gas solvents, specialized enclosure cells are sometimes needed. These contain both the sample and the cantilever, and allow measurement either in the static environment provided or with the perfusion of added liquids and gases.
With some films, the applications include the potential for corrosion and other electrochemical reactions. The use of electrochemistry cells for an AFM makes it possible to conduct processes like film deposition in situ. Other processes which can be carried out this way include electroplating, corrosion, oxidation, and mass transfer. This type of cell allows imaging for nanoscale changes in the structure due to electrochemical reactions, reflected in the topography.
.jpg)
Figure 5. Solvent vapor annealing of a polystyrene-polybutadienepolystyrene (PS-PB-PS) triblock copolymer film. Films formed by spin casting organize into microphase morphologies that do not necessarily represent the lowest energy state. Annealing often results in restructuring, and solvent vapor techniques offer advantages over standard thermal annealing. Topography images were acquired continuously as the film was exposed to toluene vapor in a closed sample cell. Scan size 2 μm, line scan rate 10 Hz. Imaged with the Cypher ES.
.jpg)
Figure 6. In-situ monitoring of zinc electrodeposition in an ionic liquid electrolyte. Zinc is an attractive material for electrodes in rechargeable batteries, but it can form detrimental morphologies that short circuit the cell. Uniform surface roughness that does not change with time reduces the probability of forming such features. These topography images show the morphology of a film grown at 325 mV deposition overpotential versus increasing film thickness (or equivalently, time). Surface roughness initially increased with thickness and time but eventually became constant (not shown). In contrast, the roughness always increased for films deposited at higher and lower overpotentials. Imaged with the MFP-3D AFM and the Electrochemistry Cell. Scan size 2 μm, height scale 200 nm. Adapted from Ref. 8.
Asylum AFMs for Assessing Thermal and Solvent Effects
- The Cypher ES AFM is an instrument designed particularly to offer precision controls of the experimental setting, with a sealed cell that can be used to expose the sample to any solvent, however harsh. It can be used under a static environment or with perfusion of required liquids or gases. Gases can be subjected to relative humidity control. The device has a heater stage up to an ambient temperature of 250 oC and a cooler/heater from 0 oC to 120 oC ambient, for exact regulation of the temperature.
- The MFP-3D AFM family uses Closed Fluid Cells for static or perfusion modes, with gas or liquid environments. The BioHeaterTM allows temperature control up to an ambient temperature of 80 oC. The device also has a Humidity Sensing Cell option if required.
- MFP-3D AFMs also have a PolyHeater to raise the ambient to 300 oC, a PolyHeater+ for ambient up to 400 oC, and CoolerHeater (from -30 oC to 120 oC) optional sample stage for temperature control.
- MFP-3D AFMs also have an Electrochemistry Cell made from chemically inert material called polyether ether ketone (PEEK) designed for robust electrochemical experiments.
|
Measuring Electrical, Electromechanical and Magnetic Functionality
A lot of applications take advantage of thin film properties, whether electrical, magnetic or electromechanical, to obtain the required level of performance. Some of these include piezoelectric actuation, pyroelectric sensors and ferroelectric nonvolatile memory. These functional aspects are not assessed directly by topographic evaluation. As a result, AFM techniques have been published to achieve this goal, as shown in Table 1.9-12
They make use of the very high sensitivity of the AFM to force and examine magnetic, electrostatic and other types of tip-sample interactions. The capability of nanoscale resolution allows both uniform or blanket type films and patterned films to be measured, as when a thin-film device has failed and analysis is proceeding. Being able to image the topography and functionality at the same time has provided great insight into how the structure is related to the properties of the material.
Some of the AFM modes used to examine electrical properties include conductive AFM or CAFM, electrostatic force microscopy or EFM, and Kelvin probe force microscopy or KPFM.9 Figure 7 shows how conductivity variations at terrace steps are detected with ease in graphene using CAFM. These modes also help evaluate the film coverage and uniform distribution. Scanning microwave impedance microscopy, or sMIM, allows the measurement of permittivity and conductivity. In Figure 8, buried structures are undergoing imaging of their electrical permittivity.
Piezoresponse force microscopy or PFM is advanced and highly adaptable for analyzing piezoelectric, ferroelectric and multiferroic films. 11 It can measure electrochemical responses in both static and dynamic settings, like polarization reversal, domain structure, and growth, as seen in Figure 2, on a nanoscale. If conventional PFM were used to measure thin films, the signal-to-noise ratio is likely to be poor unless the drive voltage is so high that the polarization would switch, or the sample might even degrade or be damaged. By using a frequency near the contact resonance frequency of the cantilever, a lower drive voltage can be used without sacrificing sensitivity.
Asylum AFMs for Electrical, Electromechanical, and Magnetic Functionality
- NanoTDDB is an exclusive Asylum mode to measure time-dependent dielectric breakdown on much smaller length scales (~20 nm) than possible with conventional probe stations. Based on CAFM, NanoTDDB involves applying a tip-sample voltage until a breakdown event is detected. The voltage can be held constant or ramped up to ±220 V or ±150 V (system dependent).
- The ORCA module provides great capabilities for CAFM on Cypher and MFP-3D family AFMs. The ORCA module features an exclusive low-noise amplifier for sensitive measurements over a wide current range (~1 pA-20 nA). The Dual Gain ORCA option allows an even wider current range (~1 pA-10 μA).
- Asylum offers the only commercial high-voltage PFM mode (up to ±220 V or ±150 V depending on system). Resonance-enhanced PFM for high sensitivity is completely integrated into software on all Asylum AFMs with the patented Dual AC™ Resonance Tracking (DART) method or the exclusive Band Excitation option.
- sMIM is a mode for scanning microwave impedance microscopy that is available integrated exclusively with MFP-3D family and Cypher S AFMs. It allows imaging of variations in electrical permittivity and resistivity on insulators, semiconductors and conductors.
- The Variable Field Module 3 for all MFP-3D family AFMs except Origin offers further versatility for studies of magnetic field dependence. It creates adjustable fields as high as ±0.75 T in plane. The exclusive design based on permanent magnets avoids heating and associated drift.
Table 1. AFM modes for functional properties. For each mode is shown its acronym as defined in the text, the physical quantity measured, and examples of information that can be acquired.
.jpg)
.jpg)
Figure 7. Electrical characterization of graphene with CAFM. The sample was a quasi-freestanding bilayer formed by chemical vapour deposition on a semi-insulating 4H-SiC (0001) (silicon carbide) substrate. The image shows CAFM current for a bias voltage of -1.5 V DC overlaid on topography. Areas with three regimes of electrical conductance can be identified: low (purple), intermediate (gold), and high (white). The step edges of the underlying substrate induce a resistance anisotropy that results in high conductance channels along the terrace steps. Scan size 10 μm, current scale 120 pA. Acquired with the Cypher S AFM and the ORCA™ CAFM module. Sample courtesy Institute of Electronic Materials Technology (ITME, Warsaw, Poland).
When magnetic behavior is to be measured at nanoscale, magnetic force microscopy or MFM12 is preferred as it takes advantage of the forces of interaction between the magnetic sample and a magnetized tip. This allows ferromagnetic and multiferroic films to be probed with higher sensitivity for functionality. Such films find application in read/write heads, spintronics and high-density data storage.
MFM is used to analyze a magnetic domain pattern or wall, a magnetic vortex, or even the lines of flux in superconductors. Similar to other AFMs, MFM is also chosen for characterization due to the high-resolution imaging capability, absence of any requirement for sample preparation, and compatibility with multiple surroundings. Figure 9 shows how MFM was used to examine the influence of film deposition variables on magnetic domains.
.jpg)
Figure 8. sMIM imaging of subsurface permittivity variations. The schematic diagram shows that the sample contained silica (SiO2 ) squares 90 nm thick buried under a thicker silicon nitride (Si3N4 ) film. After deposition of the Si3N4 film, the sample was polished so that topography variations were less than 0.5 nm. The image contains the relative capacitance signal obtained with sMIM overlaid on topography. The buried structures are clearly detected, despite little or no difference in topography. Scan size 20 μm. Acquired on the MFP-3D AFM. Image courtesy PrimeNano, Inc.
.jpg)
Figure 9. MFM evaluation of ferromagnetic FePt films grown by pulsed laser deposition (PLD). Understanding how magnetic behavior depends on PLD laser frequency could enable better control of film properties for applications such as data storage and recording. In these images, light (yellow) and dark (purple) regions represent domains polarized in the up and down directions, respectively. Overlaying the MFM phase signal on topography shows that the films deposited at 2 Hz and 6 Hz form single domain structures that correlate exactly with FePt islands. The 1 Hz film has a percolated morphology and forms multiple magnetic domains.Scan size 1 μm. Acquired with the MFP-3D AFM. Adapted from Ref. 13.
Measurement of Mechanical Characteristics
Many thin film applications require critical limits for mechanical properties such as modulus, hardness and friction. Films which are wear-resistant, for instance, enhance the properties of all kinds of products from shaving razor blades to hip replacement prostheses. Otherwise, good mechanical properties confer durability and dependability on a product. A low-k dielectric film would fracture and delaminate while it was being integrated with copper interconnects, for instance, unless it had high modulus and fracture toughness.
With its increased spatial resolution and excellent force sensitivity, the AFM can offer sophisticated nanoscale measurement of mechanical characteristics. Thus techniques based on AFM need much less application forces than conventional nanoindentation, which in turn reduces the indentation depth. This means that films of much lower thickness can be probed without creating substrate-based artifacts. Also, the good lateral resolution allows both patterned and heterogeneous films to be assessed directly.
Force curves have long been used to measure the elastic modulus.14 The relative stiffness of many materials used for films (modulus over 10 GPa) makes it difficult to produce adequate force in order to produce enough indentation when standard cantilevers are used. Conventional force mapping is too slow a technique. However, the introduction of Fast Force Mapping has speeded this process up significantly.
Two newer AFM modes called the AM-FM Viscoelastic Mapping Mode and Contact Resonance Viscoelastic Mapping Mode are both capable of achieving rapid and accurate mechanical measurements quantitatively, using stiff materials, at nanoscale. These may be employed for a rapid qualitative imaging using contrast as well as for advanced quantitative mapping, as shown in Figure 10.
Analyzing tribology characteristics at nanoscale is thus a top priority for a number of film applications. Force curves are useful for surface adhesion measurements, while lateral force microscopy (LFM) and similar techniques are used for frictional force measurement. LFM is a method in which the tip is moved laterally while maintaining contact with the sample, and then measuring how much the cantilever was deflected using the horizontal photodiode channel.
It can be employed to image any spatial differences in the sideways force, or to obtain friction loops by mapping the lateral force vs the sliding distance as shown in Figure 11. Calibrated measurements can be used while varying the applied load to find the coefficient of friction.
Get Results with Asylum AFMs
While much more may be said, enough has been revealed to show the way AFMs can be used to optimize the characterization of thin films and coatings. They can allow quantitative assessment of 3D surface roughness using unmatched spatial resolution and can map a whole range of functionalities at nanoscale. Asylum AFMs are built to remarkable technical specifications, to achieve this kind of high resolution imaging and force sensitivity, as well as incorporating other features to make their use more productive and easy, such as rapid scanning and automation of protocols.
Using Asylum AFMs to Study Nanomechanical Properties
Asylum has an array of nanomechanical measurement techniques to offer choices based on the type of application:
- The MFP-3D NanoIndenterTM is an instrument for measuring hardness and true elastic modulus (complying with ISO standards) on films over a few hundred nm thick.
- The Fast Force Mapping Mode used on the Infinity and Cypher range makes force curve mapping much simpler and reliable by taking minutes rather than hours to acquire a whole image, for instance, 256x256 pixels in less than ten minutes.
- The unique AM-FM Viscoelastic Mapping Mode of Asylum instruments allows the elastic modulus E’ to be mapped rapidly, and measurement of the viscoelastic loss tangent using old tapping mode principles. This works best for 100 KPa <E’<100+ GPa.
- The contact resonance viscoelastic mapping mode extends the reach of quantitative mapping of elastic storage modulus and viscous loss modulus for all Asylum AFMs, and is best used at 1 GPa<E’<100+ GPa
|
.jpg)
Figure 10. Elastic modulus image of a patterned titanium film on silicon, overlaid on topography. blueDrive photothermal excitation on the Cypher AFM was used for DART contact resonance imaging.Note the strong contrast between these two very high modulus materials. Scan size 25 μm.
.jpg)
Figure 11. Nanotribology of layered polymer brush-gel films. Mechanically-graded layers with both high modulus and toughness occur frequently in nature, but synthesis of an all-polymer counterpart remains challenging. As shown in the simplified schematics, twolayer films of polyacrylamide (PAAm) on silicon were created with either a brush supporting a crosslinked brush-hydrogel (“gel”) or a gel supporting a brush. LFM experiments were performed in water with different film configurations: pure brush (PAAm-0), pure gel (PAAm-1), brush-gel (PAAm-0-1), and gel-brush (PAAm-1-0). The top graph shows friction loops of lateral force versus sliding distance and the bottom, friction force versus applied load. (Curves are color-coded to film type.)The films displayed different tribological behavior, but the response was always determined primarily by the structure of the film’s outer layer where the sliding occurred. Acquired on the MFP-3D AFM with the Closed Fluid Cell. Adapted from Ref. 16.
References and Further Reading
- M. Ohring, Materials Science of Thin Films: Deposition and Structure, 2nd ed. (Academic Press, San Diego, CA, 2002).
- T. Mehmood, A. Kaynak, X.J. Dai, A. Kouzani, K. Magniez, D.R. de Celis, C.J. Hurren, and J. du Plessis, Mater. Chem. Phys.143, 668 (2014).
- A. Duk, M. Schmidbauer, and J. Schwarzkopf, Appl. Phys. Lett.102, 091903 (2013).
- B. Bhushan, “Surface Roughness Analysis and Measurement Techniques,” in Modern Tribology Handbook Vol. 1(CRC Press, Boca Raton, USA, 2001), Chap. 2.
- M. Gu, S.A. Wolf, and J. Lu, Adv. Mater. Interfaces1, 1300126 (2014).
- A.R. Damodaran, S. Lee, K. Jambunathan, S. Maclaren, and L.W. Martin, Phys. Rev. B 85, 024113 (2012).
- E.S. Gadelmawla, M.M. Koura, T.M.A. Maksoud, I.M. Elewa, and H.H. Soliman, J. Mater. Process. Technol.123, 133 (2002).
- J.S. Keist, C.A. Orme, P.K. Wright, and J.W. Evans, Electrochim. Acta152, 116 (2015).
- R. Berger, H.-J. Butt, M.B. Retschke, and S.A.L. Weber, Macromol. Rapid Commun.30, 1167 (2009).
- K. Lai, W. Kundhikanjana, M.A. Kelly, and Z.X. Shen, Appl. Nanosci.1, 13 (2011).
- S.V. Kalinin, B.J. Rodriguez, S. Jesse, E. Karapetian, B. Mirman, E.A. Eliseev, and A.N. Morozovska, Annu. Rev. Mater. Res. 37, 189 (2007).
- U. Hartmann, Annu. Rev. Mater. Res.29, 53 (1999).
- I.A. Golovchanskiy, A.V. Pan, S.A. Fedoseev, and M. Higgins, Appl. Surf. Sci.311, 549 (2014).
- H.J. Butt, B. Cappella, and M. Kappl, Surf. Sci. Rep.59, 1 (2005).
- R.W. Carpick and M. Salmeron, Chem. Rev.97, 1163 (1997).
- A. Li, S.N. Ramakrishna, P.C. Nalam, E.M. Benetti, and N.D. Spencer, Adv. Mater. Interfaces1, 1300007 (2014).
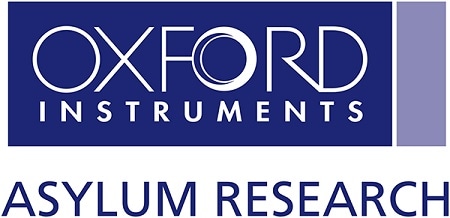
This information has been sourced, reviewed and adapted from materials provided by Asylum Research - An Oxford Instruments Company.
For more information on this source, please visit Asylum Research - An Oxford Instruments Company.