Apr 12 2022
Biosensor research has concentrated on developing a simple sensing method with rapid detection, great sensitivity, and minimum interference. Comprising a photoelectric conversion and an electrochemical process, the photoelectrochemistry (PEC) process produces an extremely sensitive and precise signal with minimal background noise.
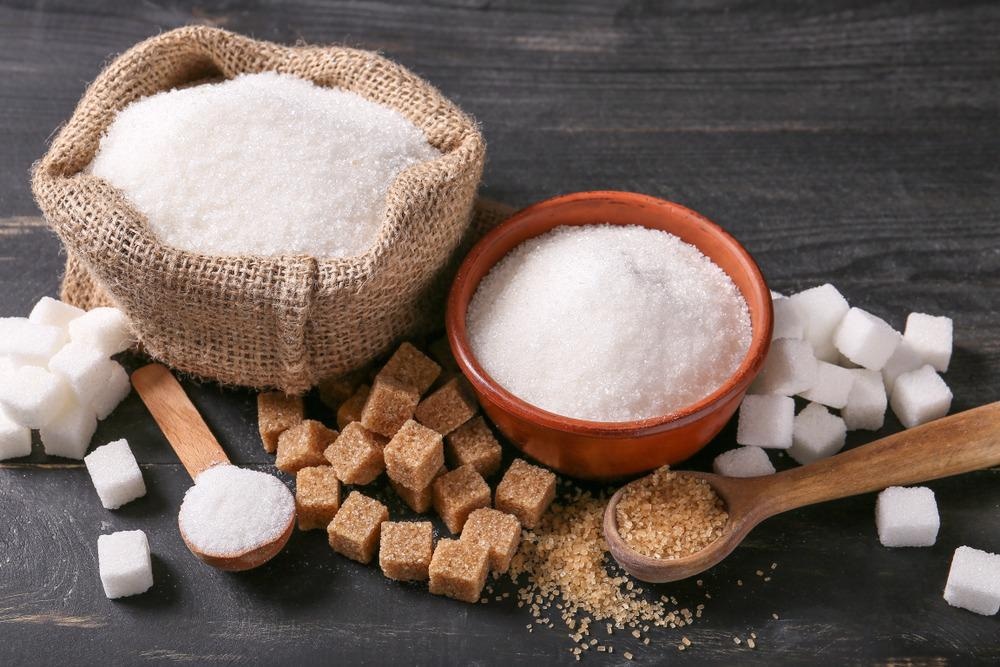
Image Credit: Pixel-Shot/Shutterstock.com
PEC-based enzyme biosensors can solve poor selectivity issues in traditional bioanalysis techniques; the photoactive material not only acts as an energy converter but also provides the target receptors’ site in PEC to precisely find and acquire the target analyte.
In research published in the journal ACS Omega, TiO2NTs/Au/Pt/GOx biosensor’s ultra-high sensitivity to glucose was achieved. The gold (Au) nanoparticles are loaded to improve the conductivity and increase the range of narrow light absorption of the material and the critical issues of electron–hole recombination.
Meanwhile, platinum (Pt) nanoparticles are deposited and employed as vital active components to catalyze the H2O2 decomposition under the enzymatic reaction. This leads to accelerated hydrogen peroxide consumption and the electrons transfer in the enzymatic reaction, thus obtaining ultra-high sensitivity for the PEC enzymatic glucose biosensor.
Results and Discussion
The PEC biosensor’s synthesis process diagram is illustrated in Scheme 1.
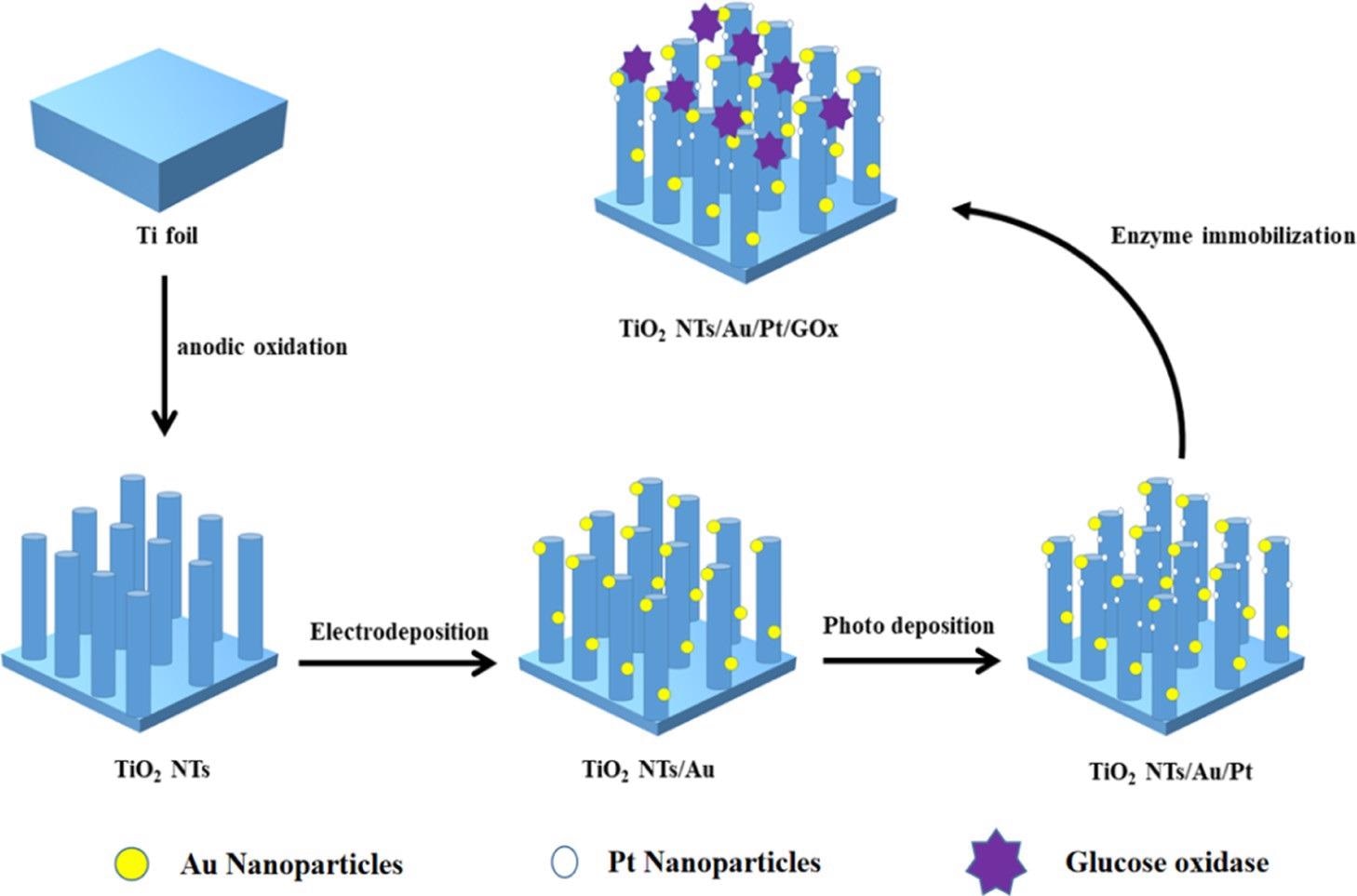
Scheme 1. Manufacturing Process of the TiO2NTs/Au/Pt/GOx Biosensor. Source: Yang, et al., 2022
Depending on the scanning electron microscopy—SEM—image (Figure 1), the prepared TiO2 nanotube film reveals a vertical one-dimensional tubular shape. The thickness and diameter of the nanotube are 20 and 80 nm, respectively. Because of the lower loading of Pt and Au nanoparticles, there was no considerable change observed on the surface of the electrode before and after the introduction of precious metals when compared with other electrodes.
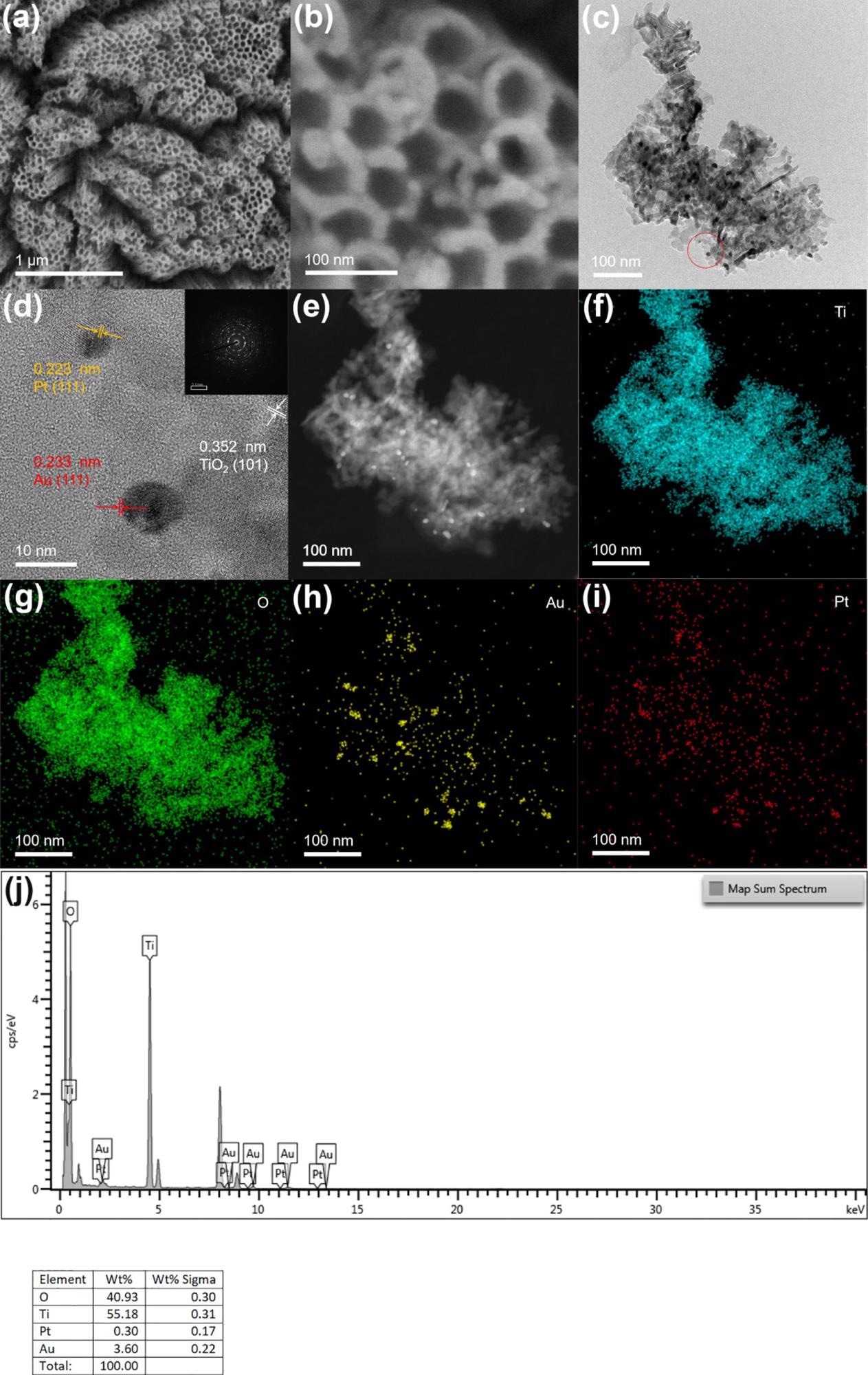
Figure 1. (b) SEM images of TiO2NTs/Au/Pt; (c,d) TEM and HR-TEM images of TiO2NTs/Au/Pt; (e–i) HAADF-STEM and mapping of Ti, O, Au, and Pt of TiO2NTs/Au/Pt; and (j) EDS spectra of TiO2NTs/Au/Pt. Image Credit: Yang, et al., 2022
The researchers analyzed the structure of the electrode material, based on XRD tests on the electrodes. The Ti foil peak is indexed to the JCPDS database (44-1294), whereas the peaks situated on 25.28, 37.8, and 48.05° are designated to the crystal facets of anatase titanium dioxide (JCPDS 21-1272) at (101), (004), and (200), respectively, as shown in Figure 2a.
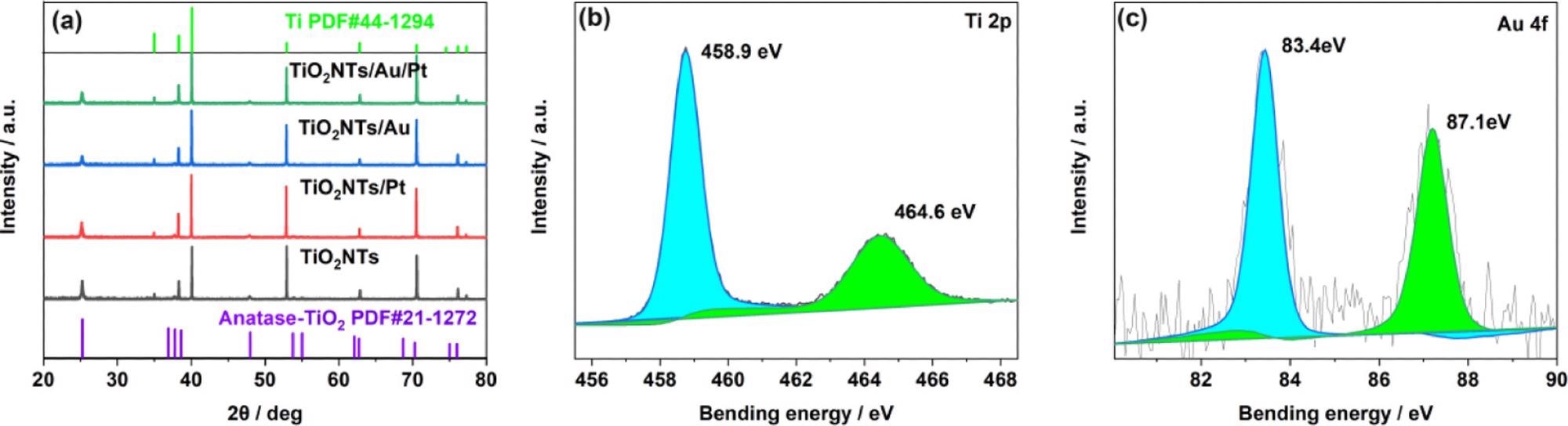
Figure 2. (a) XRD patterns of TiO2NTs, TiO2NTs/Pt, TiO2NTs/Au, and TiO2NTs/Au/Pt; (b) XPS spectra for Ti 2p; and (c) Au 4f core level for TiO2NTs/Au/Pt. Image Credit: Yang, et al., 2022
In all the four systems (TiO2NTs, TiO2NTs/Pt, TiO2NTs/Au, and TiO2NTs/Au/Pt), UV–visible absorption spectra were measured (Figure 3a). An apparent absorption cut-off edge close to the anatase maximum absorption wavelength of 387 nm is shown by all four photoelectrodes This result proves that the noble metal load does not change the TiO2 inherent absorption.
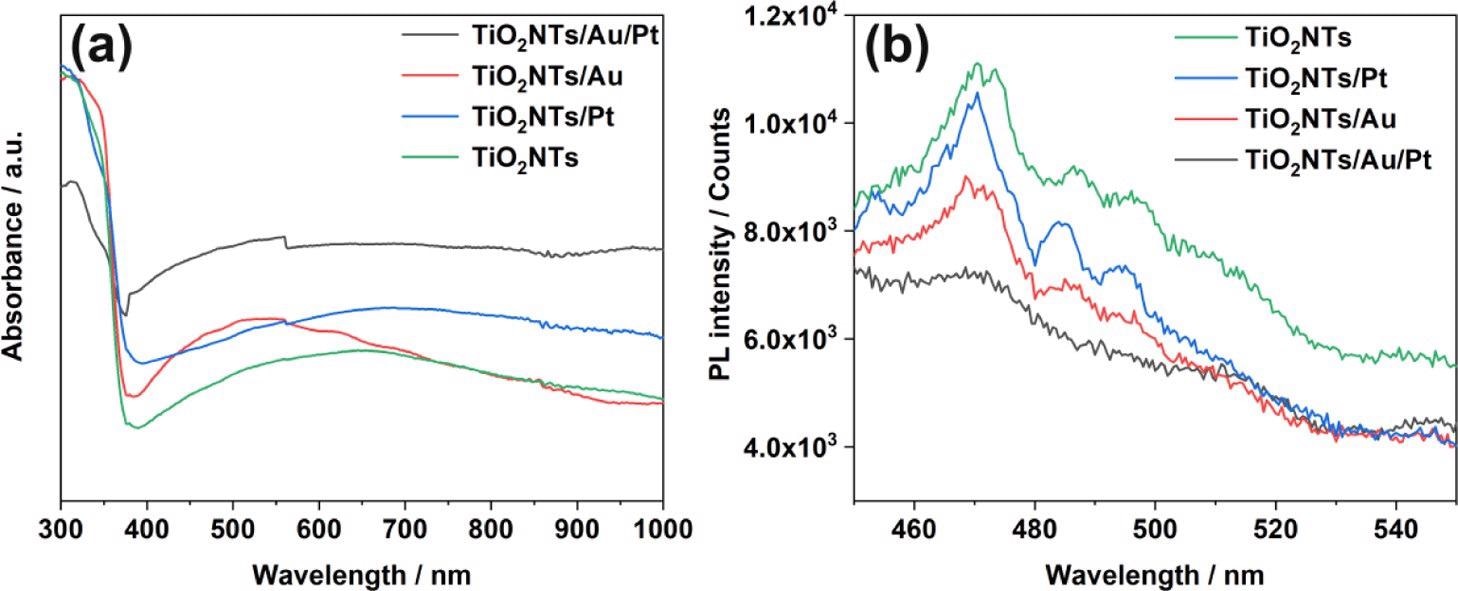
Figure 3. (a) UV–vis absorption spectra and (b) PL spectra of TiO2NTs, TiO2NTs/Pt, TiO2NTs/Au, and TiO2NTs/Au/Pt in the powder form at 350 nm excitation. Image Credit: Yang, et al., 2022
Depending on Figure 3b, the emission intensities were acquired. The pure TiO2NTs have great PL intensity, highlighting the crucial recombination of photo-generated holes and electrons.
The PEC and electrochemical experiments were performed to analyze the PEC performance of the TiO2NTs/Pt, TiO2NTs, TiO2NTs/Au, and TiO2NTs/Au/Pt electrodes. The results of PEC reveal that the photocurrent density values are seen in the order: TiO2NTs/Au/Pt > TiO2NTs/Au > TiO2NTs/Pt > TiO2NTs. The findings confirm that precious metals are advantageous for photovoltaic performance.
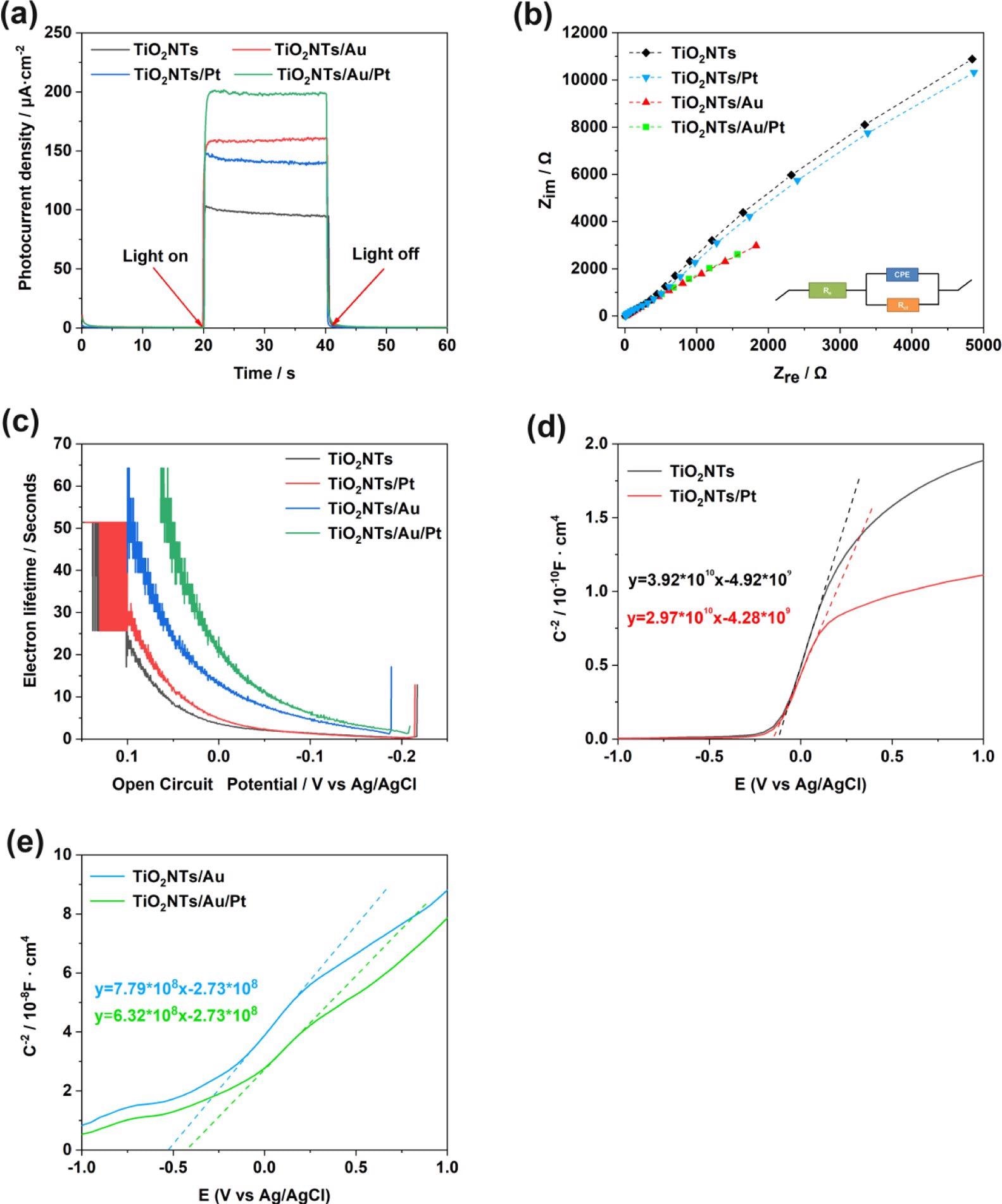
Figure 4. (a) Photoelectric response of TiO2NTs, TiO2NTs/Pt, TiO2NTs/Au, and TiO2NTs/Au/Pt under simulated sunlight (bias voltage = 0.4 V, 0.1 M PBS, pH = 7.4); (b) electrochemical impedance spectra of Nyquist plots; (c) electronic lifetime measurement determined from the decay of the open-circuit potential in the absence of light; and (d, e) Mott–Schottky plot in the dark at a fixed frequency of 5 kHz. Image Credit: Yang, et al., 2022
The current density of TiO2NTs and TiO2NTs/Au electrodes in the absence of PtNPs were elevated, while one mM hydrogen peroxide is added to the buffer.
Assuming that Pt can decompose hydrogen peroxide in the solution and produce a large number of electrical signals under the potential applied for the catalytic oxidation, the densities between the TiO2NTs/Pt and TiO2NTs/Au/Pt electrodes altered with PtNPs that varied with 12.7 and 13 μA cm–2 (vs Ag/AgCl), respectively, at 0.4 V. To improve electron transfer in enzymatic reactions, PtNPs were able to catalyze hydrogen peroxide.
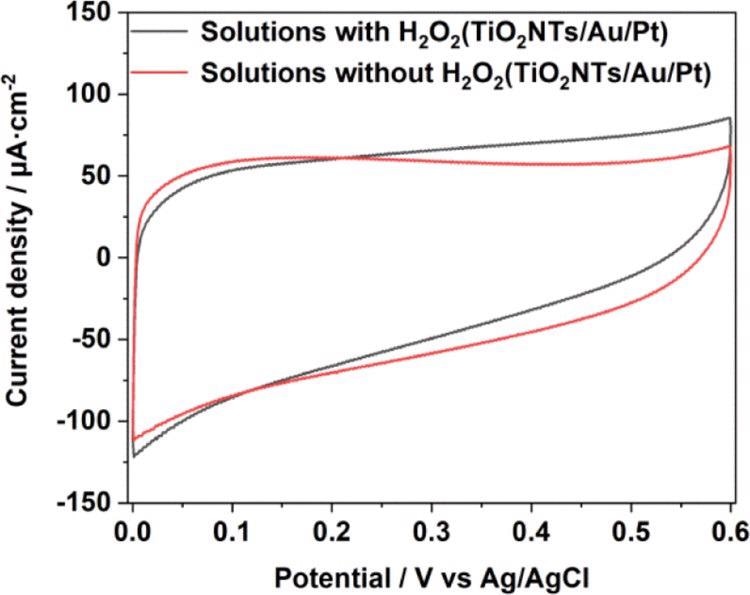
Figure 5. CV comparison in 0.1 M pH = 7.4 PBS solution with and without a H2O2 chart of TiO2NTs/Au/Pt. Image Credit: Yang, et al., 2022
The TiO2NTs/Au/Pt/GOx electrode’s photoelectric response was tested at stepped glucose concentrations. The linear regression equation between glucose concentration and the photocurrent is illustrated in Figure 6.
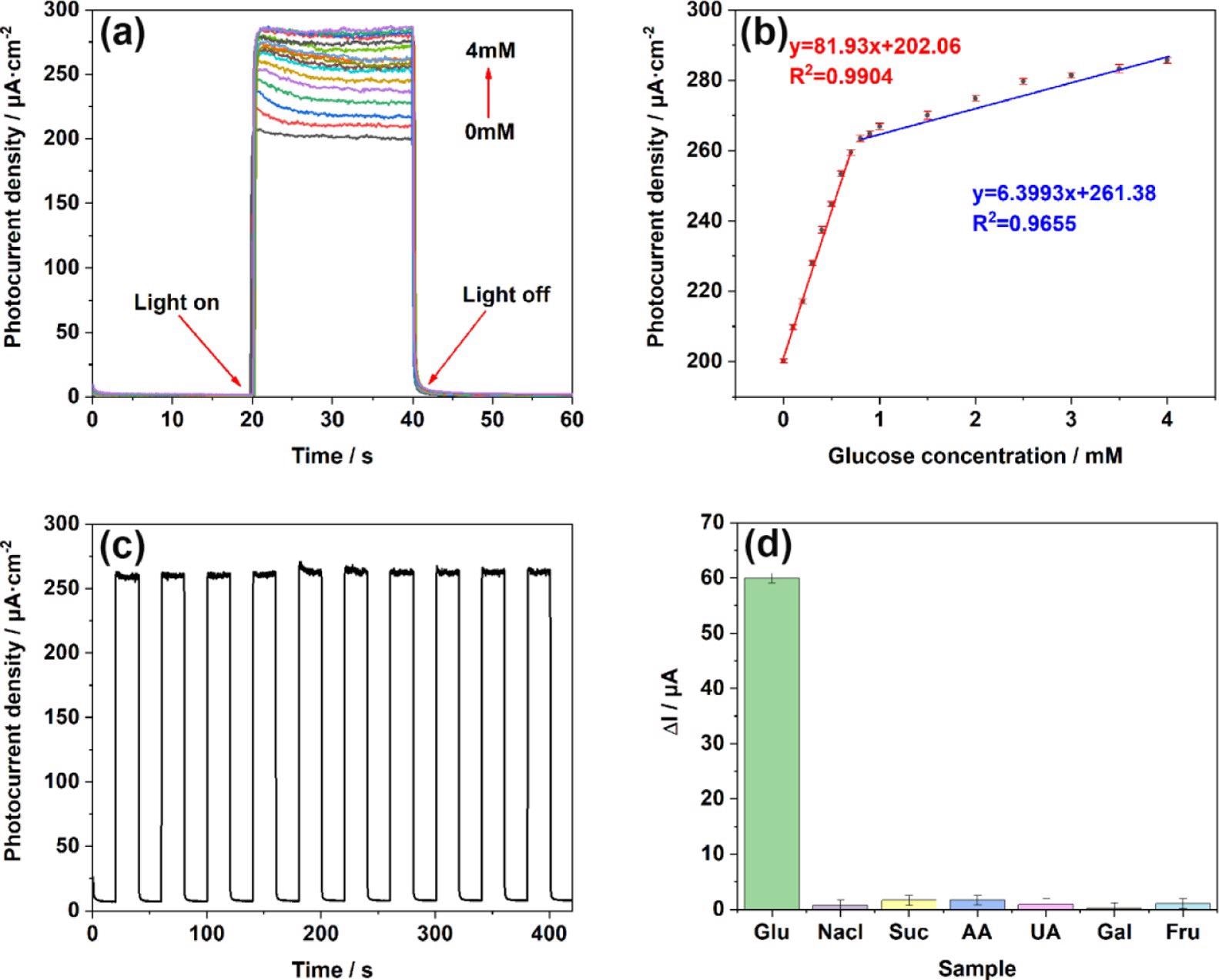
Figure 6. (a) i–t responses of TiO2NTs/Au/Pt toward Glu at concentrations of 0–4 mM in the supporting electrolyte of 0.1 M PBS (pH = 7.4); (b) linear calibration curve (glucose concentration vs photocurrent density); (c) stability after addition of 1 mM glucose under light conditions; and (d) anti-interference performance test performed in 0.1 M PBS (pH = 7.4) at concentrations of 0.1 mM chloride (NaCl), sucrose (Suc), ascorbic acid (AA), uric acid (UA), galactose (Gal), and fructose (Fru). Image Credit: Yang, et al., 2022
The researchers also showed the biosensor sensibility on the TiO2NTs/Au/Pt/GOx electrode ten times after switching the i–t response.
According to Table 1, the TiO2NTs/Au/Pt/GOx biosensor has better detection limits and higher sensitivity than the other PEC glucose biosensors.
Table 1. Comparison of the Analytical Performance of PEC Glucose Biosensors. Source: Yang, et al., 2022
PEC biosensors |
dynamic range (mM) |
LOD (μM) |
sensitivity (μA mM–1 cm–2) |
ref |
TiO2-CNT-Co3O4 |
0–4 |
0.16 |
0.3 |
(26) |
FTO/Fe2O3-NB-PDA-GDH |
0–2 |
25.2 |
7.36 |
(27) |
Nafion/GOx/Ag-Pdop@CNT/GCE |
0.05–1.1 |
17 |
3.1 |
(28) |
GOx/TCS-TiO2/chitosan/GCE |
0.005–1.32 |
2 |
23.2 |
(29) |
TiO2 NTs/GOx |
0–1, 1–4 |
61.1 |
8.53, 2.98 |
this work |
TiO2 NTs/Pt/GOx |
0–1, 1–4 |
3.07 |
20.9, 5.40 |
this work |
TiO2 NTs/Au/GOx |
0–0.7, 0.7–4 |
13.5 |
41.73, 4.73 |
this work |
TiO2 NTs/Au/Pt/GOx |
0–0.8, 0.8–4 |
1.39 |
81.93, 6.39 |
this work |
The study describes the PEC detection mechanism of glucose at enzyme modification electrodes as depicted in Scheme 2.
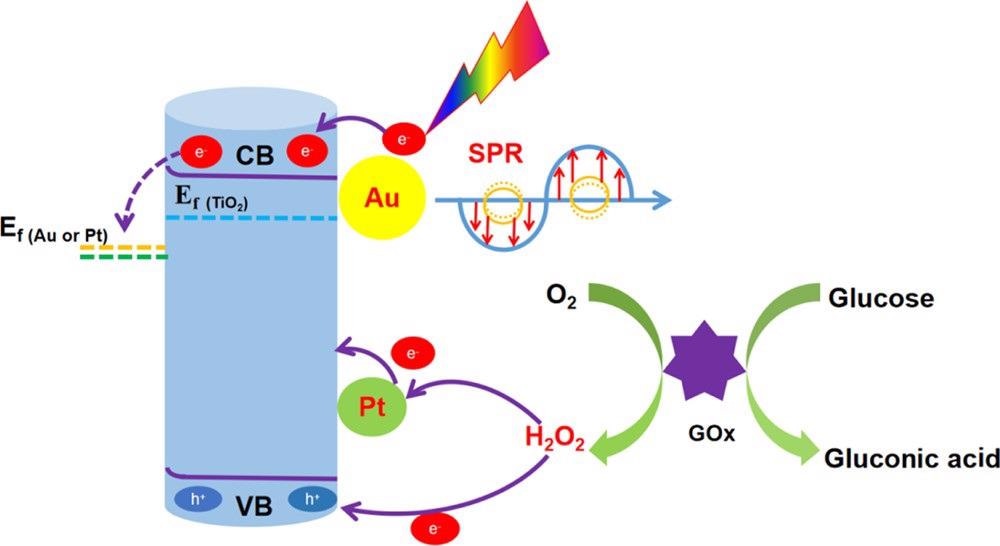
Scheme 2. Mechanism of Action of the TiO2NTs/Au/Pt/GOx PEC Biosensor for Glucose Detection. Source: Yang, et al., 2022
Methodology
Prior to the anodizing treatment, the pre-treated titanium foil sample of 10 × 20 × 0.25 mm size was polished for 30 seconds and further washed in ethanol, acetone, and deionized water for 5 minutes by ultrasonication and then dried at 50 °C in air. After which, in an electrochemical cell, anodic oxidation occurs with the working electrode being the titanium foil and the counter electrode being a stainless-steel sheet.
The electrolyte includes a glycol system comprising 6 vol % deionized water and 0.5 wt % ammonium fluoride. The specimen achieved by anodization was placed in a container and annealed in air at 400 °C for 3 hours with a two °C/min heating rate.
The precursor used was Chloroauric acid, the reference electrode used was Ag/AgCl, and the counter electrode used was Pt sheet. Gold nanoparticles were deposited electrochemically. Further, the prepared TiO2NTs/Au was cleaned with deionized water and kept to dry in a vacuum oven for 12 hours at 70 °C.
By UV lamp deposition, nanoparticles of platinum were loaded on TiO2NTs. On the target electrode, glucose oxidase is loaded by the drop coating method. Firstly, glucose oxidase was coated on the electrode surface and placed for 24 hours at four °C. Later, chitosan solution dissolved in acetic acid was provided dropwise to immobilize GOx and placed for 72 hours at four °C. Finally, TiO2NTs/Au/Pt/GOx, the target biosensor, was obtained.
Conclusions
The study efficiently designed the high-performance PEC biosensor for glucose testing with TiO2NTs/Au/Pt nanocomposites. Gold nanoparticles improved the electrode conductivity and promoted the light absorption through the SPR effect and the photogenerated carriers’ separation in the material by forming a Schottky junction. Consequently, the catalytic activity of the electrode was seen to improve greatly.
Simultaneously, PtNPs can catalyze the decomposition of H2O2 in the enzymatic reaction to improve the electrons transfer in the enzymatic reaction and further improve the PEC performance of the sensor. As a result, the achieved TiO2NTs/Au/Pt/GOx PEC biosensor has great stability and selectivity.
Journal Reference
Yang, Z., Xu, W., Yan, B., Wu, B., Ma, J., W, X., Qiao, B., Tu, J., Pei, H., Chen, D., Wu, Q., (2022) Gold and Platinum Nanoparticle-Functionalized TiO2 Nanotubes for Photoelectrochemical Glucose Sensing. ACS Omega, 7, 2, p 2474–2483. Available Online: https://pubs.acs.org/doi/10.1021/acsomega.1c06787.
References and Further Reading
- Osterloh, F E (2013) Inorganic nanostructures for photoelectrochemical and photocatalytic water splitting. Chemical Society Reviews, 42, pp. 2294– 2320, doi.org/10.1039/C2CS35266D.
- Zhang, K., et al. (2020) CoOOH nanosheets-coated g-C3N4/CuInS2 nanohybrids for photoelectrochemical biosensor of carcinoembryonic antigen coupling hybridization chain reaction with etching reaction. Sensors and Actuators B: Chemical, 307, p. 127631, doi.org/10.1016/j.snb.2019.127631.
Shu, J & Tang, D (2020) Recent Advances in Photoelectrochemical Sensing: From Engineered Photoactive Materials to Sensing Devices and Detection Modes. Analytical Chemistry, 92, pp. 363– 377. doi.org/10.1021/acs.analchem.9b04199.
Lv, S., et al. (2020) ZIF-8-Assisted NaYF4:Yb,Tm@ZnO Converter with Exonuclease III-Powered DNA Walker for Near-Infrared Light Responsive Biosensor. Analytical Chemistry, 92, pp. 1470–1476. doi.org/10.1021/acs.analchem.9b04710.
- Zhao, W.-W., et al. (2017) Photoelectrochemical enzymatic biosensors. Biosensors and Bioelectronics, 92, pp. 294– 304, doi.org/10.1016/j.bios.2016.11.009.
- Hou, T., et al. (2016) Biphasic photoelectrochemical sensing strategy based on in situ formation of CdS quantum dots for highly sensitive detection of acetylcholinesterase activity and inhibition. Biosensors and Bioelectronics, 75, pp. 359– 364, doi.org/10.1016/j.bios.2015.08.063.
- Chen, Y., et al. (2021) In situ formation of (0 0 1) TiO2 /Ti3C2 heterojunctions for enhanced photoelectrochemical detection of dopamine. Electrochemistry Communications, 125, p. 106987, doi.org/10.1016/j.elecom.2021.106987.
- Cai, G., et al. (2018) Exciton-Plasmon Interaction between AuNPs/Graphene Nanohybrids and CdS Quantum Dots/TiO2 for Photoelectrochemical Aptasensing of Prostate-Specific Antigen. ACS Sensors, 3, pp. 632– 639, doi.org/10.1021/acssensors.7b00899.
- Shu, J., et al. (2018) Plasmonic Enhancement Coupling with Defect-Engineered TiO2-x: A Mode for Sensitive Photoelectrochemical Biosensing. Analytical Chemistry, 90, pp. 2425– 2429, doi.org/10.1021/acs.analchem.7b05296.
- Sun, J., et al. (2019) Synthesis of anatase TiO2 with exposed {001} and {101} facets and photocatalytic activity. Rare Metals, 38, pp. 287– 291. doi.org/10.1007/s12598-014-0329-9.
- Hou, Y., et al. (2019) Photoeletrocatalytic Activity of a Cu2O-Loaded Self-Organized Highly Oriented TiO2 Nanotube Array Electrode for 4-Chlorophenol Degradation. Environmental Science & Technology, 43, pp. 858– 863, doi.org/10.1021/es802420u.
- Ye, M., et al. (2012) High-Efficiency Photoelectrocatalytic Hydrogen Generation Enabled by Palladium Quantum Dots-Sensitized TiO2 Nanotube Arrays. Journal of the American Chemical Society, 134, pp. 15720–15723, doi.org/10.1021/ja307449z.
- Hou, Y., et al. (2012) Role of Hydroxyl Radicals and Mechanism of Escherichia coli Inactivation on Ag/AgBr/TiO2 Nanotube Array Electrode under Visible Light Irradiation. Environmental Science & Technology, 46, pp. 4042–4050, doi.org/10.1021/es204079d.
- Mor, G. K., et al. (2007) High efficiency double heterojunction polymer photovoltaic cells using highly ordered TiO2 nanotube arrays. Applied Physics Letters, 91, p. 152111, doi.org/10.1063/1.2799257.
- Shen, S., et al. (2018) Titanium dioxide nanostructures for photoelectrochemical applications. Progress in Materials Science, 98, pp. 299– 385. doi.org/10.1016/j.pmatsci.2018.07.006.
- Melvin, A. A., et al. (2015) M-Au/TiO2 (M = Ag, Pd, and Pt) nanophotocatalyst for overall solar water splitting: role of interfaces. Nanoscale, 7, pp. 13477–13488. doi.org/10.1039/C5NR03735B.
- Wu, L., et al. (2015) Plasmon-induced photoelectrocatalytic activity of Au nanoparticles enhanced TiO2 nanotube arrays electrodes for environmental remediation. Applied Catalysis B: Environmental, 164, pp. 217– 224, doi.org/10.1016/j.apcatb.2014.09.029.
- Vaiano, V., et al. (2016) Photocatalytic removal of patent blue V dye on Au-TiO 2 and Pt-TiO 2 catalysts. Applied Catalysis B: Environmental, 188, pp. 134–146, doi.org/10.1016/j.apcatb.2016.02.001.
- Zhai, D., et al. (2013) Highly Sensitive Glucose Sensor Based on Pt Nanoparticle/Polyaniline Hydrogel Heterostructures. ACS Nano, 7, pp. 3540– 3546, doi.org/10.1021/nn400482d.
- Daniel, M. C & Astruc, D (2004) Gold Nanoparticles: Assembly, Supramolecular Chemistry, Quantum-Size-Related Properties, and Applications toward Biology, Catalysis, and Nanotechnology. Chemical Reviews, 104, pp. 293– 346, doi.org/10.1021/cr030698+.
- Tahir, M., et al. (2017) Synergistic effect in plasmonic Au/Ag alloy NPs co-coated TiO2 NWs toward visible-light enhanced CO2 photoreduction to fuels. Applied Catalysis B: Environmental, 204, pp. 548–560, doi.org/10.1016/j.apcatb.2016.11.062.
- Seh, Z., et al. (2012) Janus Au-TiO2 Photocatalysts with Strong Localization of Plasmonic Near-Fields for Efficient Visible-Light Hydrogen Generation. Advanced Materials, 24, pp. 2310– 2314. doi.org/10.1002/adma.201104241.
- Guo, N., et al. (2018) Crumpled and flexible cotton-fiber-like TiO2 with Pt anchored and its notable photocatalytic activity facilitate by Schottky junction interface. Materials Letters, 221, pp. 183–186. doi.org/10.1016/j.matlet.2018.03.131.
- Macak, J. M., et al. (2007) Efficient oxygen reduction on layers of ordered TiO2 nanotubes loaded with Au nanoparticles. Electrochemistry Communications, 9, pp. 1783– 787. doi.org/10.1016/j.elecom.2007.04.002.
- Meekins, B H & Kamat, P V (2009) Got TiO2 Nanotubes? Lithium Ion Intercalation Can Boost Their Photoelectrochemical Performance. ACS Nano, 3, pp. 3437–3446, doi.org/10.1021/nn900897r.
- Wang, G., et al. (2006) Photoelectrochemical Study on Charge Transfer Properties of TiO2–B Nanowires with an Application as Humidity Sensors. The Journal of Physical Chemistry B, 110, pp. 22029–22034. doi.org/10.1021/jp064630k.
- Ndamanisha, J C & Guo, L (2009) Nonenzymatic glucose detection at ordered mesoporous carbon modified electrode. Bioelectrochemistry, 77, pp. 60– 63. doi.org/10.1016/j.bioelechem.2009.05.003.
- Çakıroğlu, B & Özacar, M (2018) A self-powered photoelectrochemical glucose biosensor based on supercapacitor Co3O4-CNT hybrid on TiO2. Biosensors and Bioelectronics, 119, pp. 34–41. doi.org/10.1016/j.bios.2018.07.049.
- Selvarajan, S., et al. (2016) BaTiO3 nanoparticles as biomaterial film for self-powered glucose sensor application. Sensors and Actuators B: Chemical, 234, pp. 395– 403, doi.org/10.1016/j.snb.2016.05.004.
- Wang, Y., et al. (2011) Multifunctional carbon nanotubes for direct electrochemistry of glucose oxidase and glucose bioassay. Biosensors and Bioelectronics, 30, pp. 107– 111, doi.org/10.1016/j.bios.2011.08.038.
- Yang, Z., et al. (2014) Facile synthesis of tetragonal columnar-shaped TiO2 nanorods for the construction of sensitive electrochemical glucose biosensor. Biosensors and Bioelectronics, 54, pp. 528– 533. doi.org/10.1016/j.bios.2013.11.043.
- Wang, C., et al. (2017) Direct Plasmon-Accelerated Electrochemical Reaction on Gold Nanoparticles. ACS Nano, 11, pp. 5897– 5905. doi.org/10.1021/acsnano.7b01637.