Energy from various natural sources such as light, wind, and thermal energy can be transformed into measurable electrical quantities through a series of physical processes, some of which form the basis of modern technologies.
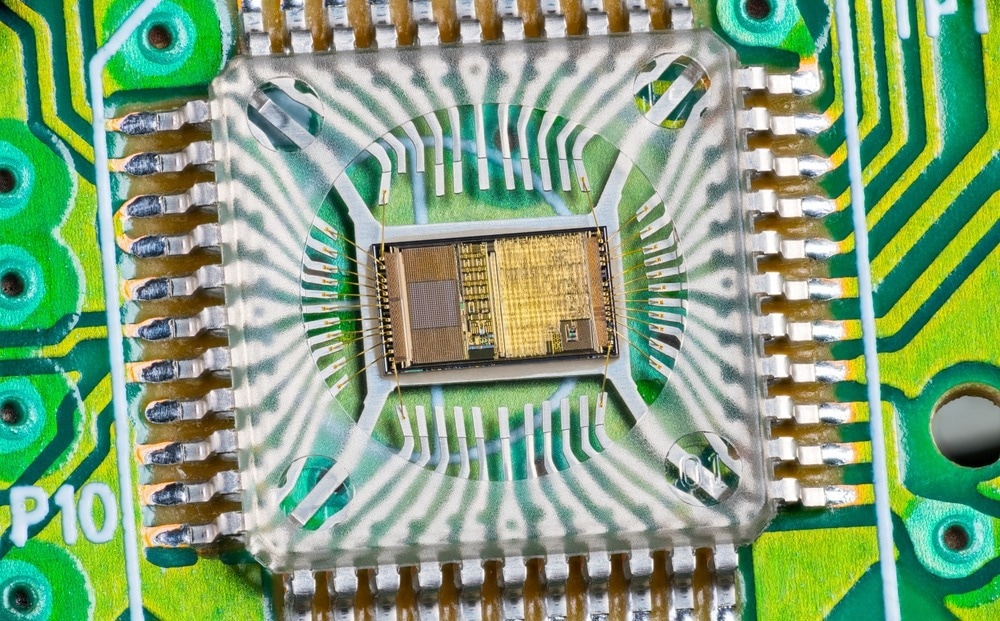
Image Credit: ShutterStock/KPixMining
Among the devices that prominently involve energy conversion, this article will focus on piezo actuators and photodiodes.
The piezoelectric effect describes the insurgence of a potential difference at two opposite sides of certain materials once a mechanical strain is applied. This is related to the fact that the deformation of the crystalline structure results in the creation of dipoles at the unit cell level.
Also, the same materials exhibit a similar effect in the opposite direction, i.e., mechanical deformation is observed when a voltage is applied to a piezoelectric sample. Piezoelectricity has a wide application in the fabrication of scanners and positioners.
Photodiodes, which are devices based on semiconductors, find practical applications as light detectors. Their operational principle relies on the fact that illuminating the surface of the photodiode with light generates a voltage proportional to the intensity of the incident light.
In cases of time-modulated light signals like sinusoidal or pulsed waves, photodiodes enable the conversion of their amplitude, frequency, and phase into electrical signals that can be analogously measured. As a result, photodiodes can facilitate the wireless transfer of both energy and information.
When considering both elements together, a device that combines the following aspects can be conceptualized: 1) receiving a light signal as input, 2) converting it into voltage, and finally, 3) initiating mechanical displacement.1 Devices that incorporate this scheme can be termed piezo-photo motion devices.
The development and analysis of such devices constitute a key focus within the research group of Professor Saive in the Department of Inorganic Materials Science (IMS) at the University of Twente, Netherlands. The potential applications of these innovations span various fields, including micro/nanorobotics for biomedical purposes.2
Atomic Force Microscopy (AFM) is thus an excellent method for studying the properties of piezo-photo motion devices, as its sensitivity to small changes in surface topography on a subnanometer scale allows it to detect even the slightest movements of these devices.
The AFM setup enables the use of Kelvin Probe Force Microscopy (KPFM), which provides information about the surface potential of samples and how it varies.
This study is the result of a collaboration between Park Systems and IMS. The goal of this article is to demonstrate how the Park Systems NX10 AFM microscope, when operated in KPFM mode, can serve as a platform for a comprehensive characterization of piezo-photo motion devices, taking into account their dependence on time and position.
The following sections will provide details about the device’s structure, the experimental setup, and the results of the KPFM experiments.
Experimental
Exemplary devices were produced and assessed at IMS. These devices consist of a silicon photodiode combined with lead zirconate titanate (PZT), a piezoelectric material, depicted as the blue layer in Figure 1a.1
The PZT actuator is situated between lanthanum nickelate (LNO) electrodes on a 50 μm silicon membrane, forming the photosensitive part of the device, as shown in the cross-section of the device.
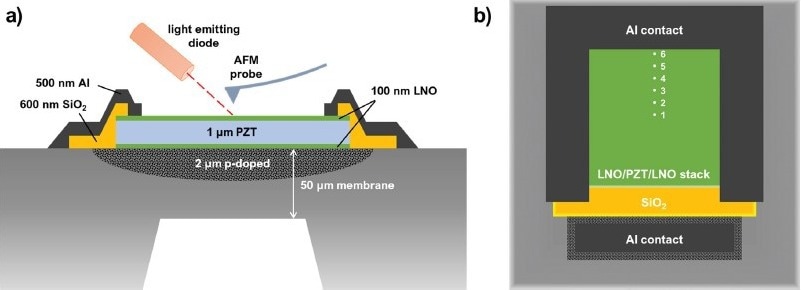
Figure 1. a) Sketch of the cross-section of the piezo-photomotion device. b) top view of the device; the numbering indicates the positions on the active area of the sample where measurements were performed.
Image Credit: Park Systems
The experiments involved inserting the devices into the NX10 microscope. Illumination was achieved using a light-emitting diode with a wavelength of around 630 nm, which is a part of the NX10 setup’s PhotoCurrent Mapping (PCM) extension.
The open design of the NX10’s AFM head provides a large space above the measurement area and thus enables a lot of freedom when it comes to optimizing the angle of the impinging beam and its position on the active area of the device.
As the LNO/PZT/LNO stack is transparent in the spectral range of the diode, the light can reach the photodiode and hence generate a potential difference between the two electrodes, which leads to the deformation of the piezoelectric layer.
Microscopes from Park Systems are designed as sample scanners, meaning the probe remains stationary at the tool’s center, while the sample on the XY scanner can be moved laterally.
Since both the light-emitting diode and the AFM head are fixed to the sample stage, a single initial alignment is sufficient to ensure the emitted beam effectively illuminates the probed sample area.
Light modulation can be achieved through a triggering voltage. An external function generator (GW Instek SFG-1003) connected to the emitter’s triggering line can alternate on/off periods of light emission at a pre-determined frequency.
The rise time of the emitter, i.e., the time taken to reach maximum light emission power, is evaluated to be less than 1 μs.
Measurements were carried out in KPFM mode, where the Contact Potential Difference (CPD) between the AFM probe and the sample is measured at each imaging position.
Various methods exist to implement KPFM, all of which involve applying an oscillating electrical bias with a specific amplitude and frequency between the tip and the sample. This electrical excitation creates peaks in the spectrum of the vertical deflection of the cantilever with an amplitude proportional to the CPD.
Using a lock-in amplifier fed with this vertical deflection as input, the amplitude can be evaluated at specific frequencies. An additional potential can then be applied to counteract the CPD and nullify the potential difference between the tip and the probe.
As a result, the deconvoluted amplitude becomes zero. An extended discussion of KPFM is outlined in reference number three.3
In Sideband™ KPFM, the frequency of the exciting bias is selected in such a way as to produce peaks on the sidebands of the mechanical resonance of the probe. As a result, peaks in that range are enhanced.
Moreover, the amplitude of these sidebands depends more on the contribution of the tip-sample interaction than on forces acting on other parts of the cantilever, leading to a better quantitative and laterally resolved determination of the potential.4
KPFM can only be conducted in Non-Contact mode while scanning the sample. Consequently, the system must possess the ability to decipher both the amplitude of the resonant peak, modulated by the sample topography and the amplitude of electrically induced peaks, modulated by the surface potential.
The default electronics of Park Systems NX come with four independent lock-in amplifiers that can operate simultaneously, along with a feedback servo that influences the tip’s DC bias.
This setup enables simultaneous mapping of topography and potential, eliminating the need for a second pass along any scanned line during lift mode. The closer the tip is to the sample, the greater the accuracy.
The built-in EFM/KPFM feature in the Park Systems SmartScan™ software allows for the effective and intuitive implementation of the Sideband™ KPFM mode.
Results
The active area of the fabricated devices is approximately square, with sides measuring a few millimeters. In the initial test, the AFM probe was positioned at the center of the membrane, marked as position 1 in Figure 1 b).
As the main focus of this test was Figure 1 a), which includes a sketch of the device’s cross-section and a top view of the device with numbered measurement positions, Figure 2 a) presents a comparison of displacement and photo-induced voltage over time using pulsed light at a switching frequency of 1 Hz.
The behaviors of the device over time at frequencies of 2 and 4 Hz are shown in Figures 2 b) and 2 c), respectively. To understand the temporal behavior of the membrane response, the scanned area was reduced to a single point, minimizing topographic interference. Signals were acquired relative to time.
Since amplitude modulation-based feedback on the tip-sample distance was active during Sideband KPFM experiments, any vertical change of the surface position induced by the movement of the membrane could have been tracked in parallel to the potential shift.
In this context, displacement is defined as the shift in surface height from its resting position.
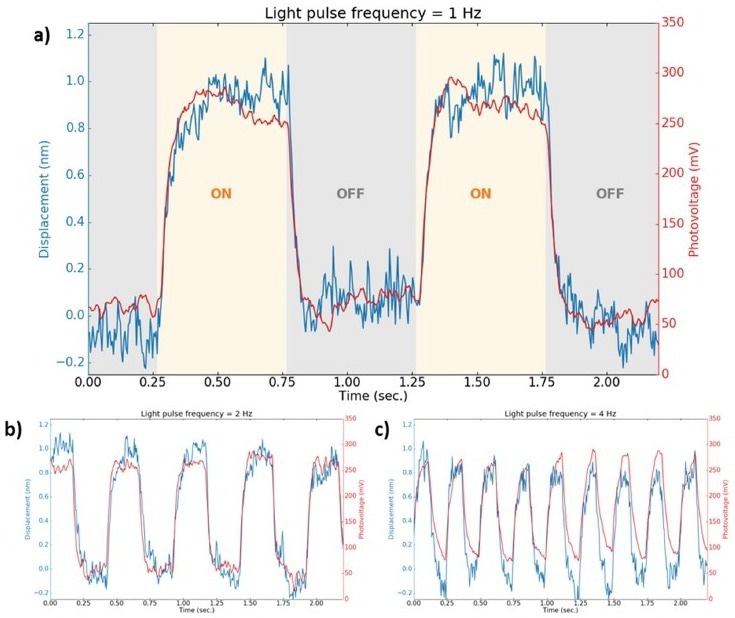
Figure 2. a) Comparison of the displacement and photo-induced voltage versus time, with a light pulsed at a switching frequency equal to 1 Hz. The device behaviors in time at frequencies equal to 2 and 4 Hz are shown in b) and c), respectively.
Image Credit: Park Systems
Figure 2 a) illustrates the outcomes of employing light excitation at a frequency of 1 Hz, alternating between 0.5 seconds of illumination and 0.5 seconds of darkness. Instances of light activation are marked with a light orange background, while the darker intervals are indicated by a light grey background.
When the light was active, there was a noticeable upward shift of approximately 1 nm. Under the same conditions, the surface potential increased by around 200 mV (defined as the difference between the upper and lower plateaus).
This shows the basic principle of the device: the voltage generated by the photodiode through illumination is directed to the lower electrode, leading to the expansion of the piezoelectric stack. The 50 mV surface potential observed during the dark phases is due to the contact potential difference between the probe and the unstimulated sample.
More tests were performed at higher pulse frequencies and results for 2 and 4 Hz are shown in Figures 2 b) and c), respectively.
A similar trend can be observed, with the total amount of displacement and photovoltage being sensibly smaller at 4 Hz. It should also be noted that the plateaux become smaller at higher frequencies, while the presence of a transient time to reach the new status after light switching becomes more apparent.
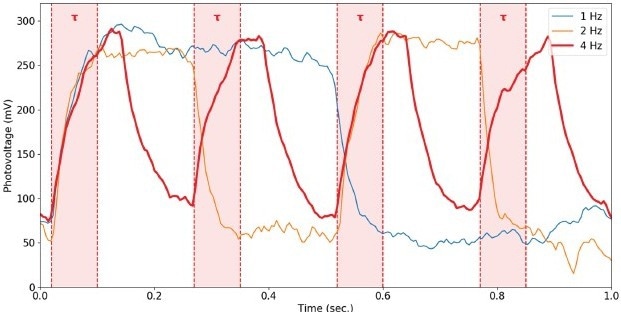
Figure 3. Photovoltage vs. time for light pulse frequency of 1, 2, and 4 Hz. The rising time of the photovoltage measured at 4 Hz is represented with a red background.
Image Credit: Park Systems
To investigate the transient response time more thoroughly, Figure 3 combines photovoltage versus time measurements. The photovoltage at a 4 Hz pulse frequency is depicted in red, spanning a total time of 1 second.
For each pulse cycle, the rise time (τ), from when the light has been switched on to when the plateau has been reached, is highlighted in red.
An estimated rise time of about 80 milliseconds is observed, notably longer than the response time of the emitting diode. This suggests that τ is a characteristic response feature of the device.
While the photovoltage stabilizes across all plotted data, a slight reduction in the difference between the levels is noticeable at a 4 Hz pulse frequency.
The cause of this occurrence remains uncertain, whether due to sustained photo response excitation at higher frequencies or other experimental factors. Until further research has been carried out to confirm this, the assumption will remain that the 80-millisecond value accurately characterizes the qualitative behavior of the response.
Photovoltage at 1 and 2 Hz pulse frequencies was also plotted, their data conveniently aligned on the x-axis for synchronized light pulse initiation.
Here, τ is consistent across all measurements.
A model to describe the time response of the device is currently under development by IMS. However, it has been hypothesized that it may be linked to the quality of the interface between the photodiode and the bottom electrode.
The experiments presented here provide crucial information about the expected performance of the device at different communication rates with respect to the exciting light source and about the efficiency in converting the transmitted signal into a movement.
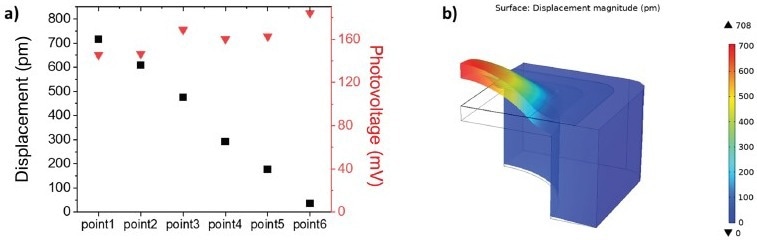
Figure 4. a) Mechanical displacement and surface potential at the positions indicated in Figure 1. b) 3D rendering of one quarter of the device representing the vertical displacement of the PZT membrane.
Image Credit: Park Systems
Using a similar device, the AFM probe approached six different positions, each progressively farther from the center. These positions were numbered, as seen in Figure 1 b). The aim was to measure how the membrane’s vertical displacement and light-induced photovoltage change with their positions on the membrane.
Light pulses with an 8 Hz frequency were used to stimulate the membrane. The results of this test are displayed in Figure 4 a).
It can be observed that the trends for displacement and photovoltage differ. For displacement, the highest value is at the membrane’s center, while it decreases to almost zero as it reaches the edge.
The center’s displacement is about 700 pm, smaller than the other device, possibly due to slight structural differences or the 8 Hz light frequency having a 62.5 millisecond on/off time, slightly less than the estimated 80 millisecond rising time. This could potentially prevent the membrane from fully settling at a displacement plateau.
In contrast, the photovoltage remains quite steady at various positions. This consistency could be due to a uniform photo response across the silicon layer at the device’s base. Additional simulations could provide more insight into these observations.
A possible explanation for the decreasing displacement with increasing distance from the center could be found in mechanical limitations, as the membrane is connected at its edges to the support. This restricts its free oscillation, leading to smaller movements.
Conclusion
This article has demonstrated the operational principle of piezo-photo motion devices. It shows how AFM, specifically using the Park Systems NX10 AFM, is ideal for studying such devices.
The article discusses KPFM measurements of light-induced displacement and photovoltage, considering their timing and positions along the device. The expected response of membrane expansion and voltage change is outlined in the article as well.
The presence of a rise time from switch-off to switch-on states is observed, which estimates the fastest rate at which the membrane can react to external light efficiently.
The article also comments on how displacement relates to membrane position, influenced by the device’s geometry, and on photovoltage response consistency.
Together, these measurements allow the investigation and improvement of piezo-photo motion and other light-driven devices.
References
- W. M. Luiten, V. M. Van Der Werf, N. Raza, and R. Saive, “Investigation of the dynamic properties of on-chip coupled piezo/photodiodes by time-resolved atomic force and Kelvin probe microscopy,” AIP Adv., vol. 10, no. 10, Oct. 2020, DOI: 10.1063/5.0028481.
- Z. Zhan, F. Wei, J. Zheng, W. Yang, J. Luo, and L. Yao, “Recent advances of light-driven micro/nanomotors: Toward powerful thrust and precise control,” Nanotechnol. Rev., vol. 7, no. 6, pp. 555–581, 2018, DOI: 10.1515/ntrev-2018-0106.
- A. Axt, I. M. Hermes, V. W. Bergmann, N. Tausendpfund, and S. A. L. Weber, “Know your full potential: Quantitative Kelvin probe force microscopy on nanoscale electrical devices”, Beilstein J. Nanotechnol., vol. 9, 1809–1819, 2018, DOI: https://doi.org/10.3762/bjnano.9.172
- J. Colchero, A. Gil, and A. M. Baro, “Resolution enhancement and improved data interpretation in electrostatic force microscopy”, Phys. Rev. B, vol. 64, 245403, 2001, DOI: 10.1103/PhysRevB.64.245403
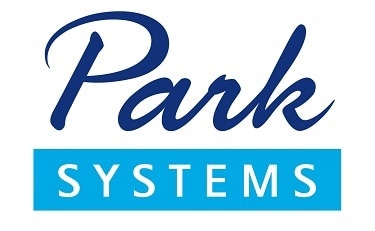
This information has been sourced, reviewed and adapted from materials provided by Park Systems.
For more information on this source, please visit Park Systems.