A 2018 FAO report found that primary crop production totaled 9.2 billion tons worldwide, up by 50% from 2000. Agricultural output, however, is heavily reliant on agrochemicals such as fertilizers and pesticides, which is unsustainable.
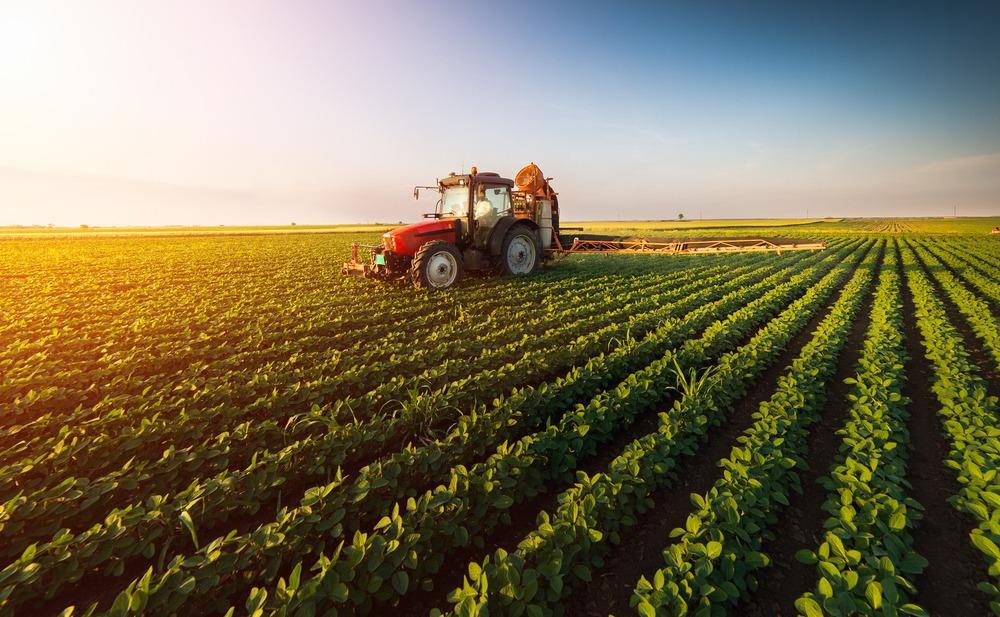
Image Credit: Fotokostic/Shutterstock.com
Overuse of fertilizers and pesticides raises agricultural product manufacturing costs while also degrading soil and polluting the environment. Furthermore, abiotic and biotic stressors are present throughout plant growth.
As a result, discovering how to help plants cope with stress from external stimulants is crucial for efficient and sustainable agriculture. It can also help to reduce the heavy reliance on pesticide products.
New strategies are essential in solving these concerns to make agricultural production more effective, durable, and sustainable.
Nanomaterials, which have at least one dimension of less than 100 nm, have been demonstrated to be promising in agriculture, particularly in enhancing plant nutrition, minimizing pests and diseases, boosting stress tolerance, and assessing plant physiological conditions.
A new review published in the journal plants, MDPI explores the sustainable agricultural applications of carbon-based nanomaterials (CNMs), particularly in nanosensors, delivery tools and light converters as shown in Figure 1.
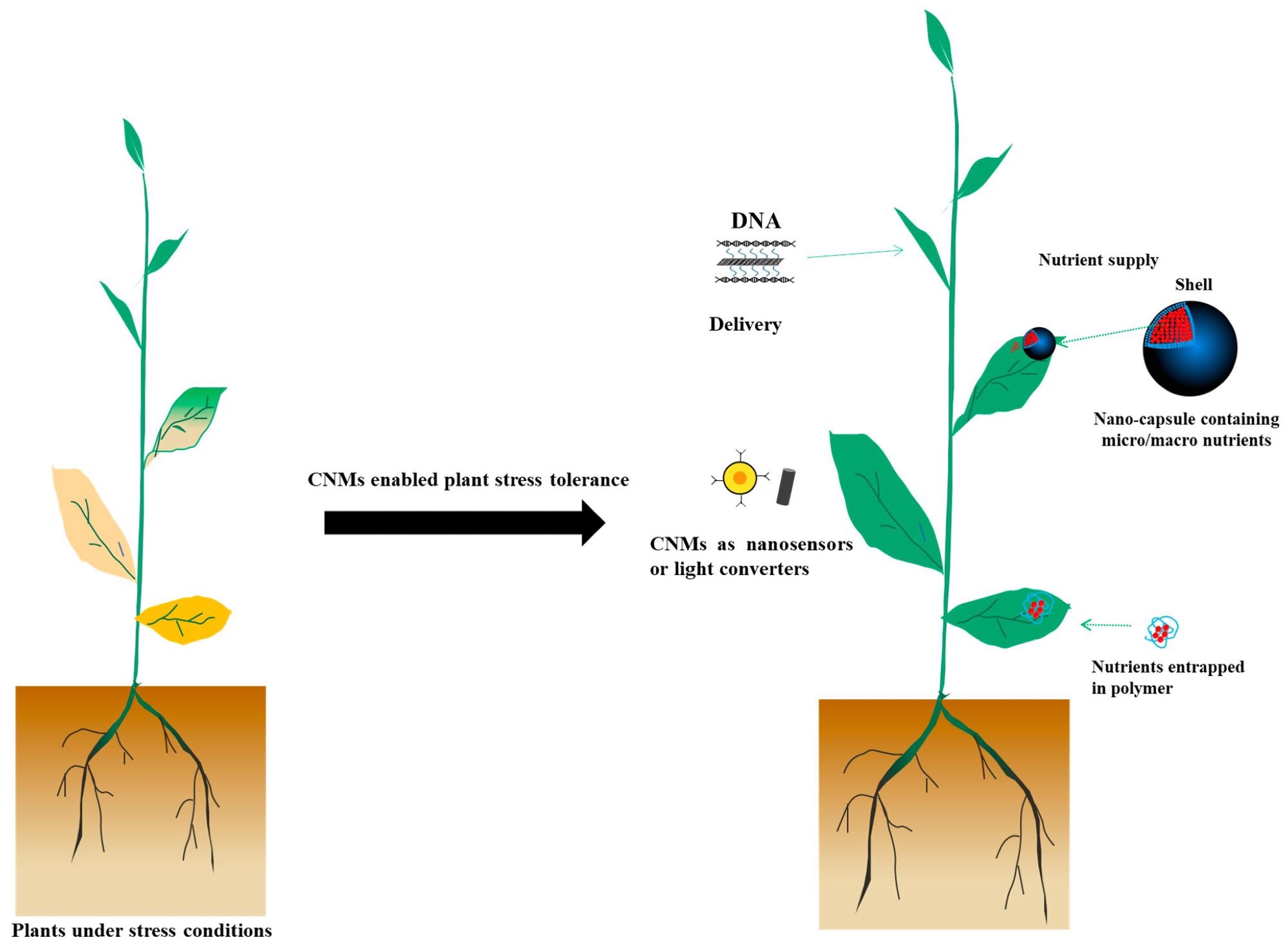
Figure 1. The role of CNMs in nanosensors, agrochemical delivery, and light converters in agriculture. Image Credit: Zhu, et al., 2022
Carbon dots (CDs), carbon nanotubes (CNTs), carbon fullerenes (C60), graphene (GRA), graphene oxide (GO), nanohorns (CNHs), and carbon nanofibers (CNFs) are all members of the CNM family.
Discussion
Remote sensing technology was created to monitor and regulate plant stress levels to alleviate the stress that leads to a decline in crop production and quality to facilitate sustainable agriculture.
Nanosensors could be a suitable choice for long-term and real-time monitoring of chemical-signaling molecules in plants, and can be used to track crop maturity and health, as well as to detect fertilizers, pesticides, and moisture in the soil, allowing farmers to make more informed decisions.
CNTs are frequently utilized in plants under abiotic and biotic stress to detect signaling chemicals such as H2O2, Ca2+, and NO, with the benefits of strong fluorescence stability, extended life, and fluorescence emission in the comparatively transparent near-infrared emission spectrum region of live tissues.
Nanosensors based on carbon nanotubes can track stress signaling molecules, allowing for better early identification of plant stress. However, most of these studies have been carried out in laboratories, and the proposed procedures have not been proven in real-world farming situations.
In pesticide detection, nanosensors based on CNMs with autofluorescence has been extensively utilized. The autofluorescence of CDs can be quenched to identify pesticides.
In conclusion, CNM-based nanosensors are promising options for assessing pesticide residues in agriculture. It is recommended that more effort be put into developing environmentally benign and biocompatible CNM-based nanosensors for detecting single or combined pesticide residues.
Agrochemicals like fertilizer and pesticides are necessary for agricultural development. However, most chemical fertilizers are used by plants less than 50% of the time, limiting the efficiency of agricultural production and polluting the environment.
Pesticides, in addition to fertilizers, are an important part of agrochemicals for agriculture. Traditional pesticides, on the other hand, have raised public concerns about their biosafety and environmental issues due to their ease of leaching, volatilization, and loss qualities.
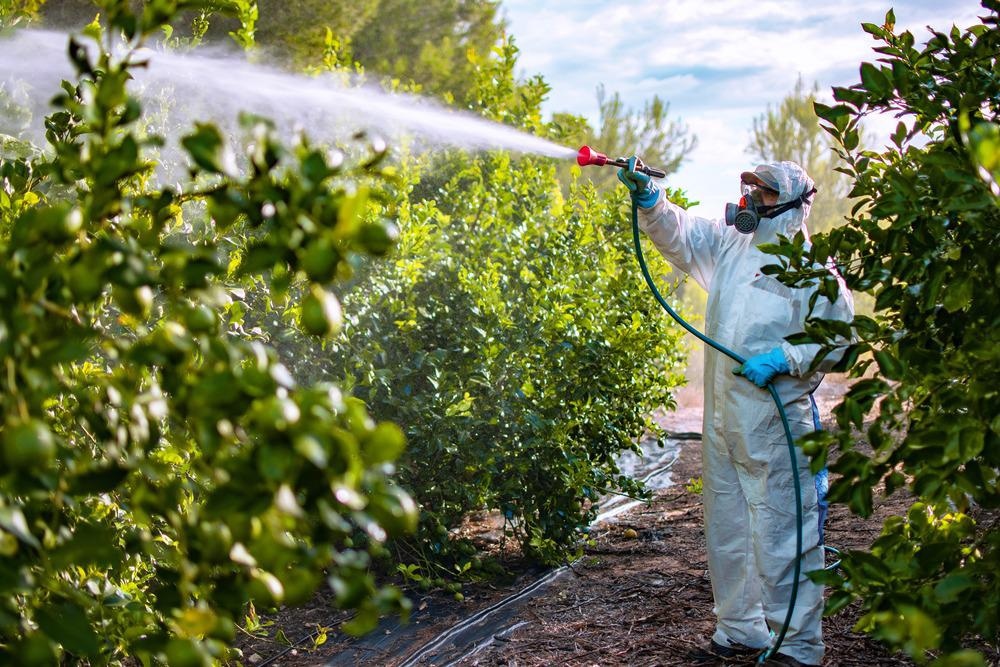
Image Credit: David Moreno Hernandez/Shutterstock.com
Excessive pesticide use has also resulted in a slew of issues that need to be addressed right once, including plant disease resistance, soil biodiversity destruction, and negative consequences on human and environmental health.
As a result, more effective and environmentally acceptable pesticide methods are advocated.
Nano-pesticides, which include nano-insecticides, nano-herbicides, and nano-fungicides, can decrease pesticide volatilization and degradation, increase pesticide utilization efficiency, reduce pesticide consumption, and reduce environmental concerns.
CNMs are especially promising and can be employed as a pesticide carrier to improve pesticide utilization efficiency, in addition to adsorbing hazardous organic matter to limit its solubility and bioavailability.
CNMs are a good option for delivering agrochemicals into plants more efficiently than traditional fertilizers and pesticides. However, it is critical to comprehend how plants react to their surroundings.
Down-conversion nanomaterials (DCNMs) are commonly utilized to convert ultraviolet (UV) light into photosynthetic active radiation.
Under UV illumination, most CDs produced today can exhibit blue fluorescence. UV light was transformed to blue light by vinyl alcohol-encapsulated CDs, which improved lettuce photosynthetic efficiency.
CDs can also be used as a UV-visible light color converter on plastic films and LEDs in greenhouses to encourage plant growth.
In conclusion, using CNMs to convert UV and nIR radiation to visible light could be a promising way to boost plant photosynthesis. However, the use of CNMs as light conversion materials in the leaves and/or roots to increase plant growth requires further research.
Conclusion
The applications of CNMs in agriculture production are discussed in this study, with the focus on their use as biosensors, carriers, and light converters. It is evident that the carbon nanoparticles might play a significant role in future agricultural output, including the improvement in food production and assistance with agricultural sustainability.
However, depending on parameters such as plant species, CNM type, and dosages, their effects may differ.
CNM research in agriculture is currently limited to the laboratory, so a considerable amount of field application data is required to allow their eventual large-scale deployment in agriculture.
Continue reading: Agricultural Applications of Nanofibers.
Journal Reference:
Zhu, L., Chen, L., Gu, J., Ma, H., Wu, H. (2022) Carbon-Based Nanomaterials for Sustainable Agriculture: Their Application as Light Converters, Nanosensors, and Delivery Tools. Plants, 11(4), p. 511. Available Online: https://www.mdpi.com/2223-7747/11/4/511/htm.
References and Further Reading
- FAO Food (2020) World Food and Agriculture Statistical Yearbook; FAO-Food: Rome, Italy.
- National Academies of Sciences, Engineering, and Medicine (2019) Science Breakthroughs to Advance Food and Agricultural Research by 2030; National Academies Press: Washington, DC, USA.
- Lowry, G.V., et al. (2019) Opportunities and challenges for nanotechnology in the agri-tech revolution. Nature Nanotechnology, 14, pp. 517–522. doi.org/10.1038/s41565-019-0461-7.
- Pretty, J (2018) Intensification for redesigned and sustainable agricultural systems. Science, 362, p. eaav0294. doi.org/10.1126/science.aav0294.
- Gogotsi, Y &Volker, P (2006) Carbon Nanomaterials; CRC Press: Boca Raton, FL, USA.
- Gohari, G., et al. (2021) Putrescine-functionalized carbon quantum dot (put-CQD) nanoparticles effectively prime grapevine (Vitis vinifera cv. ‘Sultana’) against salt stress. BMC Plant Biology, 21, p. 120. doi.org/10.1186/s12870-021-02901-1.
- Wang, X., et al. (2014) Evaluation and mechanism of antifungal effects of carbon nanomaterials in controlling plant fungal pathogen. Carbon, 68, pp. 798–806. doi.org/10.1016/j.carbon.2013.11.072.
- Li, Y., et al. (2020) A review on the effects of carbon dots in plant systems. Materials Chemistry Frontiers, 4, pp. 437–448. doi.org/10.1039/C9QM00614A.
- Xiao, A., et al. (2016) Carbon and Metal Quantum Dots toxicity on the microalgae Chlorella pyrenoidosa. Ecotoxicology and Environmental Safety, 133, pp. 211–217. doi.org/10.1016/j.ecoenv.2016.07.026.
- Bellard, C., et al. (2012) Impacts of climate change on the future of biodiversity. Ecology Letters, 15, pp. 365–377. doi.org/10.1111/j.1461-0248.2011.01736.x.
- Wu, H & Li, Z (2022) Recent advances in nano-enabled agriculture for improving plant performance. The Crop Journal, 10, pp. 1–12. doi.org/10.1016/j.cj.2021.06.002.
- Mahlein, A K (2016) Plant Disease Detection by Imaging Sensors-Parallels and Specific Demands for Precision Agriculture and Plant Phenotyping. Plant Disease, 100, pp. 241–251. doi.org/10.1094/PDIS-03-15-0340-FE.
- Fichman, Y (2019) Whole-Plant Live Imaging of Reactive Oxygen Species. Molecular Plant, 12, pp. 1203–1210. doi.org/10.1016/j.molp.2019.06.003.
- Antonacci, A., et al. (2018) Nanostructured (Bio)sensors for smart agriculture. Trac-TrAC Trends in Analytical Chemistry, 98, pp. 95–103. doi.org/10.1016/j.trac.2017.10.022.
- Giraldo, J. P., et al. (2019) Nanobiotechnology approaches for engineering smart plant sensors. Nature Nanotechnology, 14, pp. 541–553. doi.org/10.1038/s41565-019-0470-6.
- Wu, H., et al. (2020) Monitoring Plant Health with Near-Infrared Fluorescent H2O2 Nanosensors. Nano Letters, 20, pp. 2432–2442. doi.org/10.1021/acs.nanolett.9b05159.
- Huang, X., et al. (2020) A sensitive H2O2 biosensor based on carbon nanotubes/tetrathiafulvalene and its application in detecting NADH. Analytical Biochemistry, 589, p. 113493. doi.org/10.1016/j.ab.2019.113493.
- Gao, J., et al. (2014) Size-dependent impact of CNTs on dynamic properties of calmodulin. Nanoscale, 6, pp. 12828–12837. doi.org/10.1039/C4NR01623H.
- Song, H (2018) Selective Detection of NO and NO2 with CNTs-Based Ionization Sensor Array. Micromachines, 9, p. 354. doi.org/10.3390/mi9070354.
- Boghossian, A. A., et al. (2011) Near-infrared fluorescent sensors based on single-walled carbon nanotubes for life sciences applications. ChemSusChem, 4, pp. 848–863. doi.org/10.1002/cssc.201100070.
- Ren, Q.Q., et al. (2013) In vivo monitoring of oxidative burst on aloe under salinity stress using hemoglobin and single-walled carbon nanotubes modified carbon fiber ultramicroelectrode. Biosensors and Bioelectronics, 50, pp. 318–324. doi.org/10.1016/j.bios.2013.07.001.
- Tran, T. T., et al. (2020) Detection of a secreted protein biomarker for citrus Huanglongbing using a single-walled carbon nanotubes-based chemiresistive biosensor. Biosensors and Bioelectronics, 147, p. 111766. doi.org/10.1016/j.bios.2019.111766.
- Wang, H., et al. (2019) Gas Biosensor Arrays Based on Single-Stranded DNA-Functionalized Single-Walled Carbon Nanotubes for the Detection of Volatile Organic Compound Biomarkers Released by Huanglongbing Disease-Infected Citrus Trees. Sensors, 19, p. 4795. https://doi.org/10.3390/s19214795.
- Wang, H., et al. (2020) Bioelectronic Nose Based on Single-Stranded DNA and Single-Walled Carbon Nanotube to Identify a Major Plant Volatile Organic Compound (p-Ethylphenol) Released by Phytophthora Cactorum Infected Strawberries. Nanomaterials, 10, p. 479. doi.org/10.3390/nano10030479.
- Agarwal, R (2011) The International Code of Conduct on Distribution and Use of Pesticides. In Chemicals, Environment, Health; CRC Press: Boca Raton, FL, USA; pp. 211–226.
- Nsibande, S A & Forbes, P B C (2016) Fluorescence detection of pesticides using quantum dot materials–a review. Analytica Chimica Acta, 945, pp. 9–22. doi.org/10.1016/j.aca.2016.10.002.
- Dutta, R R &Puzari, P (2014) Amperometric biosensing of organophosphate and organocarbamate pesticides utilizing polypyrrole entrapped acetylcholinesterase electrode. Biosensors and Bioelectronics, 52, pp. 166–172. doi.org/10.1016/j.bios.2013.08.050.
- Song, Y., et al. (2016) A simple electrochemical biosensor based on AuNPs/MPS/Au electrode sensing layer for monitoring carbamate pesticides in real samples. Journal of Hazardous Materials, 304, pp. 103–109. doi.org/10.1016/j.jhazmat.2015.10.058.
- Wu, X., et al. (2017) Carbon quantum dots as fluorescence resonance energy transfer sensors for organophosphate pesticides determination. Biosensors and Bioelectronics, 94, pp. 292–297. doi.org/10.1016/j.bios.2017.03.010.
- Ashrafi, T. F., et al. (2020) Ultrasensitive fluorescent detection of pesticides in real sample by using green carbon dots. PLoS ONE, 15, p. e0230646. doi.org/10.1371/journal.pone.0230646.
- Liang, N., et al. (2021) Fluorescence and colorimetric dual-mode sensor for visual detection of malathion in cabbage based on carbon quantum dots and gold nanoparticles. Food Chemistry, 343, p. 128494. doi.org/10.1016/j.foodchem.2020.128494.
- Yuan, H., et al. (2016) Highly photoluminescent pH-independent nitrogen-doped carbon dots for sensitive and selective sensing of p-nitrophenol. RSC Advances, 6, pp. 15192–15200. doi.org/10.1039/C5RA26870B.
- Liu, Y., et al. (2018) Nitrogen-doped graphene quantum dots-based fluorescence molecularly imprinted sensor for thiacloprid detection. Talanta, 183, pp. 339–344. doi.org/10.1016/j.talanta.2018.01.063.
- Zhu, Y., et al. (2020) Nanozyme sensor arrays based on heteroatom-doped graphene for detecting pesticides. Analytical Chemistry, 92, pp. 7444–7452. doi.org/10.1021/acs.analchem.9b05110.
- Boruah, P. K., et al. (2021) Polydopamine functionalized graphene sheets decorated with magnetic metal oxide nanoparticles as efficient nanozyme for the detection and degradation of harmful triazine pesticides. Chemosphere, 268, p. 129328. doi.org/10.1016/j.chemosphere.2020.129328.
- Yoo, D., et al. (2019) Carbon dots as an effective fluorescent sensing platform for metal ion detection. Nanoscale Research Letters, 14, p. 272. doi.org/10.1186/s11671-019-3088-6.
- DeRosa, M. C., et al. (2010) Nanotechnology in fertilizers. Nature Nanotechnology, 5, p. 91. doi.org/10.1038/nnano.2010.2.
- Bandyopadhyay, S., et al. (2014) Multimicronutrient slow-release fertilizer of zinc, iron, manganese, and coppe. International Journal of Chemical Engineering, 2014, p. 327153. doi.org/10.1155/2014/327153.
- Kah, M., et al. (2018) A critical evaluation of nanopesticides and nanofertilizers against their conventional analogues. Nature Nanotechnology, 13, pp. 677–684. doi.org/10.1038/s41565-018-0131-1.
- Ghafariyan, M. H., et al. (2013) Effects of magnetite nanoparticles on soybean chlorophyll. Environmental Science & Technology, 47, pp. 10645–10652. doi.org/10.1021/es402249b.
- Dimkpa, C. O., et al. (2017) Nanoparticle and ionic Zn promote nutrient loading of sorghum grain under low NPK fertilization. Journal of Agricultural and Food Chemistry, 65, pp. 8552–8559. doi.org/10.1021/acs.jafc.7b02961.
- Hernández-Hernández, H., et al. (2019) Impact of selenium and copper nanoparticles on yield, antioxidant system, and fruit quality of tomato plants. Plants, 8, p. 355. doi.org/10.3390/plants8100355.
- Ye, Y., et al. (2019) Can abiotic stresses in plants be alleviated by manganese nanoparticles or compounds? Ecotoxicology and Environmental Safety, 184, p. 109671. https://doi.org/10.1016/j.ecoenv.2019.109671.
- Kabiri, S., et al. (2017) Graphene oxide: A new carrier for slow release of plant micronutrients. ACS Appl. Mater. Interfaces, 9, pp. 43325–4333. doi.org/10.1021/acsami.7b07890.
- Mahapatra, D. M., et al. (2022) Biofertilizers and nanofertilizers for sustainable agriculture: Phycoprospects and challenges. Science of The Total Environment, 803, p. 49990. doi.org/10.1016/j.scitotenv.2021.149990.
- Zulfiqar, F., et al. (2019) Munné-Bosch, S. Nanofertilizer use for sustainable agriculture: Advantages and limitations. Plant Science, 289, p. 110270. doi.org/10.1016/j.plantsci.2019.110270.
- Zhou, D. D., et al. (2016) Cotransport of graphene oxide and Cu (II) through saturated porous media. Science of The Total Environment, 550, pp. 717–726. doi.org/10.1016/j.scitotenv.2016.01.141.
- Ashfaq, M., et al. (2017) Carbon nanofibers as a micronutrient carrier in plants: Efficient translocation and controlled release of Cu nanoparticles. Environmental Science-Nano, 4, pp. 138–148. doi.org/10.1039/C6EN00385K.
- Bityutskii, N. P., et al. (2020) Fullerenol increases effectiveness of foliar iron fertilization in iron-deficient cucumber. PLoS ONE, 4, p. e0232765. doi.org/10.1371/journal.pone.0232765.
- Mew, E. J., et al. (2017) The global burden of fatal self-poisoning with pesticides 2006-15: Systematic review. Journal of Affective Disorders, 219, pp. 93–104. doi.org/10.1016/j.jad.2017.05.002.
- Rani, L., et al. (2020) An extensive review on the consequences of chemical pesticides on human health and environment. Journal of Cleaner Production, p. 124657. doi.org/10.1016/j.jclepro.2020.124657.
- Mohamed, T., et al. (2021) Vital roles of sustainable nano-fertilizers in improving plant quality and quantity-an updated review. Saudi Journal of Biological Sciences, 28, 7349–7359. doi.org/10.1016/j.sjbs.2021.08.032.
- Shakiba, S., et al. (2020) merging investigator series: Polymeric nanocarriers for agricultural applications: Synthesis, characterization, and environmental and biological interactions. Environmental Science: Nano, 7, pp. 37–67. doi.org/10.1039/C9EN01127G.
- Pan, B & Xing, B (2010) Manufactured nanoparticles and their sorption of organic chemicals. Advances in Agronomy, 108, 137–181.
- Chen, G.C., et al. (2011) Adsorption of diuron and dichlobenil on multiwalled carbon nanotubes as affected by lead. Journal of Hazardous Materials, 188, pp. 156–163. doi.org/10.1016/j.jhazmat.2011.01.095.
- Fan, X., et al. (2018) Multiwall carbon nanotubes modulate paraquat toxicity in Arabidopsis thaliana. Environmental Pollution, 233, pp. 633–641. doi.org/10.1016/j.envpol.2017.10.116.
- Maliyekkal, S. M., et al. (2013) T. Graphene: A reusable substrate for unprecedented adsorption of pesticides. Small, 9, pp. 273–283. doi.org/10.1002/smll.201201125.
- Wang, X., et al. (2019) Graphene oxide as a pesticide delivery vector for enhancing acaricidal activity against spider mites. Colloids and Surfaces B: Biointerfaces, 173,pp. 632–638. doi.org/10.1016/j.colsurfb.2018.10.010.
- Tong, Y., et al. (2018) Adhesive and Stimulus-Responsive Polydopamine-Coated Graphene Oxide System for Pesticide-Loss Control. Journal of Agricultural and Food Chemistry, 66, pp. 2616–2622. doi.org/10.1021/acs.jafc.7b05500.
- Wang, Y., et al. (2020) Polylactic Acid-Graphene Oxide-based Materials for Loading and Sustained Release of Poorly Soluble Pesticides. Langmuir, 36, pp. 12336–12345. doi.org/10.1021/acs.langmuir.0c02320.
- Yeh, C. N., et al. (2014) On the origin of the stability of graphene oxide membranes in water. Nature Chemistry, 7, pp. 166–170.0020. doi.org/10.1038/nchem.2145.
- Song, S., et al. (2019) Carboxymethyl Chitosan Modified Carbon Nanoparticle for Controlled Emamectin Benzoate Delivery: Improved Solubility, pH-Responsive Release, and Sustainable Pest Control. ACS Applied Materials Interfaces, 11, pp. 34258–34267. doi.org/10.1021/acsami.9b12564.
- Sarlak, N., et al. (2014) Synthesis of nanopesticides by encapsulating pesticide nanoparticles using functionalized carbon nanotubes and application of new nanocomposite for plant disease treatment. Journal of Agricultural and Food Chemistry, 62, pp. 4833–4838. doi.org/10.1021/jf404720d.
- Kah, M (2015) Nanopesticides and nanofertilizers: Emerging contaminants or opportunities for risk mitigation? Frontiers in Chemistry, 3, p. 64. doi.org/10.3389/fchem.2015.00064.
- Krenek, P., et al. (2015) Transient plant transformation mediated by Agrobacterium tumefaciens: Principles, methods and applications. Biotechnology Advances, 33, pp. 1024–1042. doi.org/10.1016/j.biotechadv.2015.03.012.
- Wang, H., et al. (2014) High-yield sorting of small-diameter carbon nanotubes for solar cells and transistors. ACS Nano, 8, pp. 2609–2617. doi.org/10.1021/nn406256y.
- Pierrat, P., et al. (2015) Efficient in vitro and in vivo pulmonary delivery of nucleic acid by carbon dot-based nanocarriers. Biomaterials, 51, pp. 290–302. doi.org/10.1016/j.biomaterials.2015.02.017.
- Schwartz, S. H., et al. (2020) Carbon Dots for Efficient Small Interfering RNA Delivery and Gene Silencing in Plants. Plant Physiology, 184, pp. 647–657. doi.org/10.1104/pp.20.00733.
- Demirer, G. S., et al. (2019) Carbon nanotube-mediated DNA delivery without transgene integration in intact plants. Nature Protocols, 14, pp. 2954–2971. doi.org/10.1038/s41596-019-0208-9.
- Demirer, G. S., et al. (2020) Carbon nanocarriers deliver siRNA to intact plant cells for efficient gene knockdown. Science Advances, 6, p. e0495. doi.org/10.1126/sciadv.aaz0495.
- Kwak, S. Y., et al. (2019) Chloroplast-selective gene delivery and expression in planta using chitosan-complexed single-walled carbon nanotube carriers. Nature Nanotechnology, 14, pp. 447–455. doi.org/10.1038/s41565-019-0375-4.
- Golestanipour, A., et al. (2018) Gene Delivery to Tobacco Root Cells with Single-Walled Carbon Nanotubes and Cell-Penetrating Fusogenic Peptides. Molecular Biotechnology, 60, pp. 863–878. doi.org/10.1007/s12033-018-0120-5.
- Scholes, G. D., et al. (2011) Lessons from nature about solar light harvesting. Nature Chemistry, 3, pp. 763–774. doi.org/10.1038/nchem.1145.
- Shevela, D & Bjorn, L.O (2018) Photosynthesis: Solar Energy for Life; World Scientific Publishing: Singapore.
- Pessarakli, M (2018) Handbook of Photosynthesis; CRC Press: Boca Raton, FL, USA.
- Duan, P., et al. (2017) Protection of photosynthetic algae against ultraviolet radiation by one-step CeO2 shellization. Langmuir, 33, pp. 2454–2459. doi.org/10.1021/acs.langmuir.6b04421.
- Wang, Y., et al. (2021) Fluorescent carbon-dots enhance light harvesting and photosynthesis by overexpressing PsbP and PsiK genes. Journal of Nanobiotechnology, 19, p. 260. doi.org/10.1186/s12951-021-01005-0.
- Liu, X. Y., et al. (2018) Photosynthesis and leaf development of cherry tomato seedlings under different LED-based blue and red photon flux ratios. Photosynthetica, 56, pp. 1212–1217. doi.org/10.1007/s11099-018-0814-8.
- Ren, J., et al. (2020) Dual-emitting CsPbX3@ ZJU-28 (X = Cl, Br, I) composites with enhanced stability and unique optical properties for multifunctional applications. Chemical Engineering Journal, 391, p. 123622. doi.org/10.1016/j.cej.2019.123622.
- Liang, L., et al. (2019) ntense broad-band absorption and blue-emitting Ca9La (PO4)5 (SiO4)Cl2: Eu2+ phosphor under near-ultraviolet excitation. Journal of Luminescence, 206, pp. 154–157. doi.org/10.1016/j.jlumin.2018.10.036.
- Nawkar, G. M., et al. (2013) UV-induced cell death in plants. International Journal of Molecular Sciences, 14, 1608–1628. doi.org/10.3390/ijms14011608.
- Wang, Y., et al. (2017) onjugated polymer nanoparticles to augment photosynthesis of chloroplasts. Angewandte Chemie International Edition, 56, pp. 5308–5311. doi.org/10.1002/anie.201702376.
- Choi, Y., et al. (2018) Carbon Dots: Bottom-Up Syntheses, Properties, and Light-Harvesting Applications. Chemistry Asian Journal, 3, pp. 586–598. doi.org/10.1002/asia.201701736.
- Li, Y., et al. (2021) Magnesium-nitrogen co-doped carbon dots enhance plant growth through multifunctional regulation in photosynthesis. Chemical Engineering Journal, 442, p. 130114. doi.org/10.1016/j.cej.2021.130114.
- Song, H., et al. (2019) High production-yield solid-state carbon dots with tunable photoluminescence for white/multi-color light-emitting diodes. Science Bulletin, 64, pp. 1788–1794. doi.org/10.1016/j.scib.2019.10.006.
- Wang, B., et al. (2019) Near-infrared emissive carbon dots with 33.96% emission in aqueous solution for cellular sensing and light-emitting diodes. Science Bulletin, 64, pp. 1285–1292. doi.org/10.1016/j.scib.2019.07.021.
- Qian, T., et al. (2018) Comparative study of single-walled carbon nanotubes and graphene nanoplatelets for improving the thermal conductivity and solar-to-light conversion of PEG-infiltrated phase-change material composites. ACS Sustainable Chemistry & Engineering, 7, pp. 2446–2458. doi.org/10.1021/acssuschemeng.8b05335.
- Edison, T. N. J. I., et al. (2020) Facile hydrothermal synthesis of nitrogen rich blue fluorescent carbon dots for cell bio-imaging of Candida albicans. Process Biochemistry, 88, pp. 113–119. doi.org/10.1016/j.procbio.2019.10.003.
- Li, L., et al. (2020) On-off-on” detection of Fe3+ and F−, biological imaging, and its logic gate operation based on excitation-independent blue-fluorescent carbon dots. Spectrochimica Acta Part A: Molecular and Biomolecular Spectroscopy, 227, p. 117716. doi.org/10.1016/j.saa.2019.117716.
- Cheng, Z., et al. (2019) Highly selective fluorescent visual detection of perfluorooctane sulfonate via blue fluorescent carbon dots and berberine chloride hydrate. Spectrochimica Acta Part A: Molecular and Biomolecular Spectroscopy, 207, pp. 262–269. doi.org/10.1016/j.saa.2018.09.028
- Xu, X., et al. (2020) PVA-coated fluorescent carbon dot nanocapsules as an optical amplifier for enhanced photosynthesis of lettuce. ACS Sustainable Chemistry & Engineering, 8, pp. 3938–3949. doi.org/10.1021/acssuschemeng.9b07706.
- Chandra, S., et al. (2014) High throughput electron transfer from carbon dots to chloroplast: A rationale of enhanced photosynthesis. Nanoscale, 6, pp. 3647–3655.doi.org/10.1039/C3NR06079A.
- Li, W., et al. (2018) Enhanced Biological Photosynthetic Efficiency Using Light-Harvesting Engineering with Dual-Emissive Carbon Dots. Advanced Functional Materials, 28, p. 1804004. doi.org/10.1002/adfm.201804004.
- Li, Y., et al. (2021) Carbon dots as light converter for plant photosynthesis: Augmenting light coverage and quantum yield effect. Journal of Hazardous Materials, 410, p. 124534. doi.org/10.1016/j.jhazmat.2020.124534.
- Guner, T., et al. (2019) Optimization and performance of nitrogen-doped carbon dots as a color conversion layer for white-LED applications. Beilstein Journal of Nanotechnology, 10, pp. 2004–2013. doi.org/10.3762/bjnano.10.197.
- Zhang, X., et al. (2020) High-efficient, spherical and thermal-stable carbon dots@ silica fluorescent composite as rare earth-free phosphors for white LED. Ceramics International, 46, pp. 14706–14712. doi.org/10.1016/j.ceramint.2020.02.274.
- Cao, M., et al. (2019) A yellow carbon dots-based phosphor with high efficiency for white light-emitting devices. Journal of Luminescence, 206, pp. 97–104. doi.org/10.1016/j.jlumin.2018.10.056.
- Barman, B. K., et al. (2020) Dual roles of a transparent polymer film containing dispersed N-doped carbon dots: A high-efficiency blue light converter and UV screen. Applied Surface Science, 510, p. 145405. doi.org/10.1016/j.apsusc.2020.145405.
- Ma, Q., et al. (2022) Photovoltaic/spectrum performance analysis of a multifunctional solid spectral splitting covering for passive solar greenhouse roof. Energy Conversion and Management, 251, p. 114955. doi.org/10.1016/j.enconman.2021.114955
- Shah, S. A. A., et al. (2021) Recent advances and emerging trends of rare-earth-ion doped spectral conversion nanomaterials in perovskite solar cells. Journal of Rare Earths, in press.
- Cheng, Z., et al. (2013) Multiwalled carbon nanotubes and NaYF4:Yb3+/Er3+ nanoparticle-doped bilayer hydrogel for concurrent NIR-triggered drug release and up-conversion luminescence tagging. Langmuir, 29, pp. 9573–9580. doi.org/10.1021/la402036p.
- Zhang, Y., et al. (2017) facile ultrasonic-assisted fabrication of nitrogen-doped carbon dots/BiOBr up-conversion nanocomposites for visible light photocatalytic enhancements. Scientific Reports, 7, p. 45086. doi.org/10.1038/srep45086.
- Xu, X., et al. (2020) Promoting the growth of mung bean plants through uptake and light conversion of NaYF4: Yb, Er@ CDs nanocomposites. ACS Sustainable Chemistry & Engineering, 8, pp. 9751–9762. doi.org/10.1021/acssuschemeng.0c02024.
- Garfield, D. J., et al. (2018) Enrichment of molecular antenna triplets amplifies upconverting nanoparticle emission. Nature Photonics, 12, 402–407. doi.org/10.1038/s41566-018-0156-x.
- Miyako, E., et al. (2014) Photofunctional nanomodulators for bioexcitation. Angewandte Chemie, 126, pp. 13337–13341. doi.org/10.1002/ange.201407169.
- Nakatsuji, H., et al. (2015) Thermosensitive Ion Channel Activation in Single Neuronal Cells by Using Surface-Engineered Plasmonic Nanoparticles. Angewandte Chemie International Edition, 54, pp. 11725–11729. doi.org/10.1002/anie.201505534.