This Graphene Battery User’s Guide, which has been created for both scientists and non-scientists, explains the working principle of graphene batteries, their benefits, and details immediate, actionable steps that can be taken to begin developing a graphene battery.
Don’t miss out on the next phase of nano evolution. This guide covers four well-established graphene electrode designs of experiments (DOE) from innovative academic research, including one for a graphene-lithium-sulfur battery, the current front runner technology, and are included for reference.
.jpg)
The molecular structure of a graphene sheet
Graphene-based batteries are being actively studied for a variety of commercial applications. The improved performance and life cycle benefits when developing graphene-based batteries over conventional metal-ion batteries are well worth the resource investment.
Elon Musk’s Tesla Motors provides a well-known example of innovative companies actively involved in graphene battery research and commercialization.
The real graphene battery breakthroughs are expected from graphene-lithium-ion hybrid chemistries incorporated into the cathodes of lithium-sulfur cells. This kind of technology will take many years to be commercialized and intensive research is ongoing. The more advanced graphene battery technologies will need considerable R&D expenditures and will take many years to commercialize.
Traditional Battery Technology
.jpg)
A cross sectional view of a traditional battery
Lithium is the common material used in both rechargeable and non-rechargeable batteries. Although alkaline- and zinc-based batteries are available, they typically have a shorter service life because of their high charge density. Unlike lithium-based batteries, these batteries cannot operate at higher voltages.
A primary (non-rechargeable) battery is composed of two electrodes, allowing current flow in one direction only, via an intermediary electrolyte. Secondary (rechargeable) batteries still contain two electrodes, but lithium ions can flow in both directions depending on if charging or discharging.
The anode is typically a lithium-based (metal oxide) compound and the cathode is a porous carbon. Both the anode and cathode have a rigid structure with defined holes, enabling the absorption of lithium ions into the holes upon the application of current. The ions desorb into the electrolyte solution when there is no current being applied.
Absorption of the lithium ions can take place on both the cathode and the anode. The ions move towards to the cathode when a battery is being used. During charging, the current is reversed and the ions are absorbed into the anode. This process allows for many cycles to be produced, resulting in an enhanced lifespan. The material of choice for cathodes is traditionally graphite, but it can vary for anodes. The most common types include Li4Ti5O12, LiNiCoAlO2, LiFePO4, LiNiMnCoO2 (NMC), LiCoO2, and LiMn2O4.
Graphene Battery Technology
.jpg)
Graphene batteries can reduce the environmental impact of battery use
The structure of graphene battery technology is similar to that of traditional batteries, where two electrodes and an electrolyte solution are used to facilitate ion transfer. The main difference between graphene-based batteries and solid-state batteries is in the composition of one or both electrodes.
The change primarily lies in the cathode, but it is also possible to utilize carbon allotropes in the anode. The cathode in a conventional battery is purely composed of solid-state materials, but a composite-a hybrid material containing a solid-state metallic material and graphene is used as the cathode in a graphene battery.
Depending on the intended application, the amount of graphene in the composite can differ. The amount of graphene incorporated into the electrode is usually based on the performance requirements and depends upon the existing efficiencies and/or weaknesses of the solid-state precursor material.
Graphene Battery Breakthrough
The real graphene battery breakthroughs are the incorporation of graphene-lithium-ion hybrid chemistries into the cathodes of lithium-sulfur cells as described in this guide. Pure graphene electrodes are not used in a graphene battery, and many graphene-based electrodes are fabricated and operate in a similar way to conventional batteries.
Their performance is improved by adding graphene to the electrode formulation. Inorganic-based electrodes generally have limitations in terms of density, conductivity, surface area, capacity, capacitance, or cycle times to name a few.
Graphene is a versatile molecule possessing many unique and desirable properties, and it can be adopted in many different ways, as there is no ‘one size fits all’ solution for using graphene. Graphene is used to improve many of the benefits already present with conventional materials, but it also helps to overcome previous battery limitations, resulting in increased battery life or performance.
In electrodes, graphene works in two general ways, either as a composite/hybrid or as a support material. As a support material, graphene helps to keep metal ions in a regular order, which helps with electrode efficiency.
As a composite material in an electrode, graphene is generally involved in the facilitation of the charge itself, where its high conductivity and well-ordered structure are important attributes to provide an improvement against its non-graphene predecessors. The role of graphene in enabling certain battery applications is discussed in the following sections.
Lithium-Ion Batteries
Graphene-based batteries are rapidly becoming more favorable compared to their graphite predecessors. They are an emerging technology, allowing for faster cycle times and increased electrode density. They also have the ability to retain the charge longer, improving the lifespan of the battery.
Graphite batteries are a proven technology and available in many forms. Like graphite, various types of functional graphene derivative electrodes are now available and researchers are discovering many advantages over pure graphite electrodes.
Graphene-Metal Oxide Hybrids
Historically, graphite has been employed as the primary cathode material, where the lithium ions move into the structured holes. However, graphene lacks this capability, but it can store the lithium ions via surface adsorption and induced bonding due to its large surface area.
Induced bonding generally occurs during the presence of a graphene derivative and the lithium ions attach to the functionalized surface. In addition to possessing a large surface area, high conductivity is another key characteristic of graphene electrodes. Many metal oxides traditionally used in batteries have downsides such as low volumetric energy density, low conductivity, and the loss of contact points.
Hybridizing the metal oxide matrix with graphene can eliminate most of these problems, and the conductivity becomes greater due to vast improvement in the interaction between the interstitial ions and the hybrid matrix. To produce graphene-metal oxide nanoparticle hybrids, graphene serves as a template during the process, producing an evenly distributed matrix owing to the regular repeating structure of graphene.
This process also limits the aggregation of nanoparticles, promoting the large nanoparticle surface area during the lithium charge and discharge cycles. As such, there is an improvement in the specific capacity and cycling performance compared to pure MO-based electrodes.
Graphene-MO hybrid electrodes are capable of exhibiting up to 1100 mAh g-1 for the first 10 cycles. The specific energy density is maintained at 1000 mAh g-1, even after 130 cycles.
Graphene-Carbon Nanotube/Fullerene Hybrids
While not as widely reported, an electrode containing both graphene and either carbon nanotubes and/or fullerenes have been implemented. Electrodes consisting of 6-15 monolayers of graphene, with each layer on top of one another, exhibit a specific capacity of 540 mAh g-1, which is considerably higher when compared to their graphitic counterparts due to their higher surface area.
Dispersing the graphene sheets with either fullerenes or carbon nanotubes increases the inter-graphene spacing. This extra spacing forms extra cavities for the lithium ions to occupy, increasing the specific capacity by up to 40% compared with stacked graphene electrodes.
Graphene Lithium Sulfur Batteries
.jpg)
A graphene-lithium-sulfur battery
Lithium sulfur batteries have the potential to substitute lithium-ion batteries in commercial applications, due to their low toxicity, low cost, and the potential for possessing an energy density of 2567 W h kg-1, which is five times higher than that of existing lithium-based batteries.
As such, they have gained a lot of interest, but have several significant shortcomings that have prevented them from reaching the commercial marketplace. The first is inorganic salt deposition at the cathode due to the presence of highly soluble reactants in the cell.
The salt deposition causes a loss in active material, low utilization of the sulfur cathode, low coulombic efficiency, and a degradation of the cycle life. The second major drawback is the inherent low conductivity of sulfur.
Using graphene as a support material for the sulfur ions eliminates some of the associated problems in sulfur batteries, thanks to its unique properties, such as a large surface area, high chemical/thermal stability, good mechanical strength, and high electrical conductivity.
The high surface area provides a good dispersion of sulfur, which controls the mobilization of sulfur ions and eliminates a build-up of sulfur ions on the cathode. A one-pot synthesis can also be used to produce graphene supported sulfur particles.
The performance of lithium-sulfur batteries relies largely on sulfur concentration (and effectively the graphene to sulfur ratio) in the electrode. From electrodes produced to date, electrodes containing higher sulfur content deliver the best performance. Electrodes consisting of 63 wt% sulfur have a capacity of 731 mAh g-1, with the potential of obtaining up to 1160 mAh g-1.
The capacity remains at 700 mAh g-1 even after 50 cycles and is at least double the capacity of other sulfur-graphene electrodes fabricated. They are also stable under high rate cycling. Some degradation of the cell does occur because the sulfur binding has not yet achieved 100% efficiency, but graphene supported sulfur electrodes still demonstrate a vast improvement when compared with non-graphene-based sulfur electrodes.
Graphene Supercapacitors
.jpg)
A graphene supercapacitor design
In the electronics field, supercapacitors are a useful device capable of storing up more than a hundred times more energy than standard capacitors. They can also work in low temperature conditions and are regularly used as a replacement for electrochemical batteries.
The ability to produce double-electric layers is one of the key properties of a supercapacitance material, and is important in electric double-layer capacitors (EDLC) supercapacitors. Supercapacitors store energy by building up charges at the electrode-electrolyte interface through polarization.
Activated carbon has been traditionally used as the electrode material, but the inability to work at high voltages is its major disadvantage. Graphene, and its derivatives, are useful due to their open-pore structure, high conductivity, high specific surface area, production potential and low cost; all of which are desirable attributes for a supercapacitor.
Graphene-Metal Oxide Composite Electrodes
.jpg)
Graphene molybdenum oxide electrodes
Graphene and metal oxide composite electrodes have provided a new area wherein the capacitance is higher than that of standard capacitor electrodes. Common metal oxides are MnO2, Co3O4, SnO2, and ZnO2. MnO2 graphene composites are the most promising due to the variable oxidation states that manganese ions can adopt.
The redox reaction between the III and IV states involves the intercalation of metal ions in the electrolyte solution (e.g. Li+, Na+), which facilitates energy storage. In addition to the efficient ion intercalation, the graphene sheets provide a conductive network with a large surface area, which further promotes ion-electrode interactions.
Graphene-MO composites possess a high specific capacitance of 310 F g-1, which is three times more than an electrode made of either pure graphene or metal oxide. They can retain 95.6% of its specific capacitance even after 2000 cycles.
As previously mentioned, supercapacitors are currently preferred over electrochemical batteries for certain applications. However, lower energy density is an issue with using supercapacitors.
The incorporation of graphene into the negative electrodes of supercapacitors increases the energy density, while keeping the power density high. Supercapacitors have been made using a nanowire-graphene composite as the positive electrode and graphene as the negative electrode.
This capacitor is configured asymmetrically and has been shown to deliver a high-energy density of 30.4 Wh kg-1 at an operating voltage of 2 V. The graphene supercapacitors have outperformed other capacitors in terms of power density and energy, making them promising in the field of supercapacitors.
Graphene-Polymer Composite Electrodes
.jpg)
A graphene-polymer composite electrode
Graphene-polymer composites do not have high conductivity compared to other graphene-based composites, but they do possess a high doping-undoping capability, high charge/discharge rate, and flexibility. Graphene-polymer composites work by n and p doping redox reactions where electrons are lost or gained to convert and store energy.
Graphene oxide and a nitrogen containing polymer are ideal to fabricate a graphene-polymer electrode composite. Polymerization of the functional groups facilitates strong pi-pi interactions between the two components of the composite, resulting in a large surface area and a semi-flexible structure that can mechanically deform during the cycle charge-discharge processes. These graphene-polymer composites can exhibit up to 531 F g-1 and retain up to 74% of its capacitance even after 2000 cycles.
Graphene-CNT Electrodes
.jpg)
A representation of aligned carbon nanotubes in between graphene sheets
Similar to lithium-ion batteries, carbon nanotubes are used to increase the inter-graphene spacing between the different monolayers. Stacked graphene electrodes can suffer from a reduced surface area compared to monolayered graphene. The introduction of nanotubes into the electrode increases the nanopores as well as the conductivity of the electrode.
Nanotubes can be used into the graphene matrix in either two or three-dimensions, with an average specific capacitance of 120 F g-1 and 386 F g-1, respectively. The capacitance can even rise by up to 20% after 2000 cycles in the three-dimensional graphene-CNT, demonstrating the excellent electrochemical stability of these electrodes.
Graphene Fuel Cells
.jpg)
A fuel cell inside view
Unlike batteries and capacitors, fuel cells generate electricity rather than store it. Many fuel cells consist of a platinum-based catalyst, which is very expensive to produce. The cost of fuel cells can be lowered using carbon allotropes as a support for the platinum catalysts. One such catalyst support is graphene.
With a large surface area, good dispersion, and high conductivity (when reduced) of graphene oxide, the integrity and efficiency of fuel cells is improved by incorporating graphene into the composite materials used in the device.
Graphene is employed in some fuel cells to facilitate the oxidation of methanol and has been shown to be more effective when compared with other carbon allotropes, such as carbon black and carbon nanotubes. Two-dimensional graphene sheets possess a greater active surface area for electron/ion transport because both of their sides are exposed to the solution within the fuel cell.
The uniformity of the graphene surface also eliminates aggregation, promoting an even distribution of the platinum particles across the supports. Also, the surface defects in the graphene structure promote the interaction between the graphene support and the platinum particles. Platinum-graphene supported fuel cells can have a current density up to 0.12 mA cm-2, which is at least three times more than other carbon-based supports.
Graphene can be doped with nitrogen after subjecting it to a nitrogen plasma treatment. Doped graphene possesses nitrogen-based functional groups on its surface, which enable a better dispersion and decoration by the platinum nanoparticles. Doped graphene possesses a greater conductivity and electrocatalytic activity than undoped graphene. The oxidation current of doped graphene is two times that of undoped graphene.
Design of Experiments- Synthesizing a Graphene-Based Battery Electrode
There are many methods available to convert graphene and metal-based inorganic compounds into usable graphene composites for electrodes. As this is an emerging field, new methods are frequently being developed and subsequently published. Some of the methods include chemical reduction, ex-situ hybridization, electroless deposition, in-situ crystallization, hydrothermal methods, sol-gel methods, thermal evaporation, electrochemical deposition, and in-situ self-assembly.
As with any method, there are multiple ways to synthesize the material itself. To cover each approach would be impractical, so here some specific ways to implement graphene into composite materials for use as electrodes in graphene battery R&D projects are discussed.
The values used are from published experiments and are used for ratio illustrative purposes only. The scale and amounts can be varied to better suit specific experiments.
The following is a DOE for graphene-lithium-sulfur batteries, a current leading technology.
“Synthesis of the Thermally Exfoliated Reduced Graphene. Thermally exfoliated reduced graphene was obtained.
Preparation of Graphene-Sulphur Hybrids- The G/S hybrids were prepared by hydrothermal reduction assembly of GO with a sulfur-dissolving CS2 and alcohol solution. In brief, 50 mL of the GO aqueous dispersion and 15 mL of alcohol were mixed, and then 3 mL of CS2 containing 100, 150, and 200 mg of dissolved sulfur (tuning the sulfur content in the samples) was added to the GO dispersion. The mixture was stirred for 90 min and then sealed in an 80 mL Teflon-lined stainless steel autoclave for a hydrothermal reaction at 180 C for 10 h. The black cylinder of the G/S hydrogel was washed by ethanol and distilled water, and the wet hydrogel was then freeze-dried to obtain the G/S hybrids.
Preparation of Graphene-Sulphur Hybrids (Powder)- G/S hybrids (powder) were prepared by mixing 90 mg of intercalation-exfoliated graphene and thermally exfoliated reduced graphene with 150 mg of sulfur under the same hydrothermal conditions as the G/S hybrids.
Preparation of Graphene-Sulphurmix The G/Smix was prepared by mixing- 50 mL of the GO aqueous dispersion, 15 mL of alcohol, and 150 mg of sulfur under the same conditions but without CS2.
Electrochemical Measurements. The G/S hybrid was cut, compressed, and shaped into a circular pellet with a diameter of 12 mm and directly used as a cathode. The mass loading of a G/S electrode was about 2 mg cm2. The G S59 or G S60 hybrid (powder) cathode was prepared by mixing 90 wt % G S59 or G S60 hybrid (powder) with 10 wt % polyvinylidene fluoride dissolved in N-methyl-2-pyrrolidone as a binder to form a slurry, which was then coated on an aluminum foil and dried under vacuum at 70 C for 12 h. The foil was pressed between twin rollers, shaped into a circular pellet with a diameter of 12 mm, and used as a cathode. The electrolyte was 1.0 M lithium bis-trifluoromethanesulfonylimide in 1,3-dioxolane and 1,2-di- methoxyethane (1:1 by volume) with 0.5 wt % LiNO3 additive. A 2025 type stainless steel coin cell was used to assemble a test cell. A lithium metal foil was used as the anode, and a G/S slice as the cathode. A LAND galvanostatic charge discharge instrument was used to perform the measurements. The coin-type cell was assembled in an Ar-filled glovebox (MBraun Unilab). The current density set for cell tests was referred to the mass of sulfur in the cathode and varied from 0.3 to 4.5 A g-1. The charge discharge voltage range was 1.5 2.8 V. The CV was measured using a VSP-300 multichannel potentiostat/galvanostat (Bio-Logic, France) workstation in the voltage range 1.5 2.8 V (vs Liþ/Li) at a scan rate of 0.1 mV s-1. The G S63 hybrid electrode was discharged to the end of the second plateau and disassembled, dried in the glovebox, and followed by transferring to the vacuum chamber of XPS for structure characterization.”1
Three more types of graphene electrode DOEs are discussed in the following sections.
A pure graphene-based electrode is created by dispersing graphene oxide powder (100 mg) in distilled water (30 mL) and sonicating for 30 minutes. The resultinf suspension is heated on a hot plate until it reaches 100 °C and 3 mL of hydrazine hydrate is then added.
The suspension is kept at 98 °C for 24 hours to reduce the graphene oxide to rGO. The reduced graphene oxide can be collect by filtration to leave a black powder. The filtered powder is then washed several times with distilled water in order to reduce the excess hydrazine.
The graphene powder is re-dispersed into water by sonication and the resulting solution is then centrifuged at 4000 rpm for 3 minutes to remove the larger particles. Graphene is collected by vacuum filtration and dried in a vacuum. This step can be left out if rGO is used.
To create the electrode, graphene is dispersed in ethanol until a concentration of 0.2 mgmL-1 is achieved. The resulting suspension is filtered by vacuum filtration and then collected on the microporous filter paper. The filtered graphene is cut into 1 x 2 cm2 (1 mg weight) for subsequent use. It is attached to a cell with an electrolyte buffer in order to examine the graphene electrode.
The second method explains the preparation of a cobalt-graphene hybrid electrode for use as an electrode in lithium-ion batteries. To prepare the electrode, graphene oxide (0.1 g) is added to cobalt acetate (350 mg) and deionized water (400 mL). NH4OH (3800 µL) and hydrazine (250 µL) are added to the solution and the mixture is stirred for 4 hours at 100 °C.
The resulting solution is filtered out once the reaction is complete. The solution is then re-crystallized by heating the product for 6 hours at 200 °C.
This last method involves creating a tin-graphene nanoribbon composite electrode for use in lithium-ion batteries. To create the electrode, graphene nanoribbon (GNR) (75 mg), SnCl2.H2O (1.33g, 5.89 mmol), 2-pyrrolidinone (65 mL) and a magnetic stirrer bar are added to a dried round-bottom flask.
The solution is sonicated for 20 minutes and then refluxed for 1 hour. The vessel is cooled down to room temperature and sonicated overnight in an open-air environment. The mixture is quenched with acetone and water three times, and filtered over a PTFE membrane (0.45 µm). The resulting product is dried in a vacuum (60 °C) for 24 hours and annealed in a quartz furnace (500 °C, Ar atmosphere) for 2 hours. The theoretical yield is 380 mg.
Graphene Products Available
Cheap Tubes Inc offers a variety of graphene-based products that can be used in electrode formulations.
.jpg)
Cheap Tubes’ plasma exfoliated graphene nanoplatelets
Graphene nanoplatelets (GNPs) possess some of the best properties for battery applications. They can be used as an alternative to other carbon-based materials. GNPs have outstanding thermal and electrical conductivity, mechanical stability, and the ability to provide a composite with enhanced mechanical strength, conductivities, and lower gas permeation.
Cheap Tubes produces research grade graphene nanoplatelets by plasma exfoliation. The company’s plasma exfoliation process synthesizes high-grade GNPs with a higher internal conductivity and less defects. The GNPs contain several graphene layers and typically have a thickness range of 3 - 10 nm and are friable with high shear methods such as a 3 roll mill or homogenizer.
The GNPs are produced with varying functional groups, including oxygen-based, nitrogen-based, fluorine and amine groups. Non-functionalized GNPs (argon processed) are also available. As the production methods of GNPs are scalable, these materials can be implemented in large volume and larger scale applications.
Graphene oxide (and reduced graphene oxide) is supplied as a dispersion, a powder, or a spin coated film. The elemental composition of Cheaptubes graphene oxide includes 35 - 42% carbon, 45 - 55% oxygen and 3 - 5% hydrogen. The company provides dispersions in various solvents and in a range of concentrations.
It also offers graphene oxide (and reduced graphene oxide) films and coatings. A graphene oxide film composed of Cheap Tubes’ single layer graphene oxide product spin coated on glass offers a final thickness of 5 - 20 nm. Its conductivity is in the range of 104 - 105 Sm-1 and sheet resistance in the range of 101 - 103 Ω sq-1.
Conversely, a single (flexible) graphene sheet on a flexible organic substrate offers the same thickness and area, but has a conductivity range of 103 - 104 Sm-1 and sheet resistance range of 102 - 104 Ω sq-1.
Cheap Tubes also offers CVD graphene films grown on a range of substrates, including silicon, quartz, PET, and copper. They can also be transferred to a range of customer supplied substrates if they are compatible with the solvents employed to float the graphene off the substrate so that it can be re-deposited onto the substrate.
Conductive carbon nanotube composite additives can be used as an alternative to graphene. They have been specifically designed to enhance the efficiency of lithium-ion electrodes. They are a blend of both nanotubes and carbon black, improving the tap density (up to 10%) and capacitance retention of the electrode without decline after multiple charge/discharge cycles.
The additive consists of multi-walled carbon nanotubes blended with a proprietary carbon black and can be used in the anode as well as in the cathode within a battery cell. Typically, 1-2 wt% is used in an anode and 2-3 wt% in a cathode.
Conclusion
In terms of efficiency, graphene-based batteries are quickly becoming comparable to conventional solid-state batteries. Thanks to the continuous advancements in graphene-based batteries, it will not be long before they outperform their solid-state predecessors.
The additional advantages associated with graphene being present in the electrodes can be useful, even if the efficiency is not as high. Graphene batteries are an ideal option for batteries that possess a similar efficiency and because of this reason researchers are striving to further advance this class of batteries.
Graphene batteries have started to gain interest in the commercial marketplace and it will not be long before these batteries become the norm and phase-out solid-state batteries.
To quote recent forecasts “the world graphene battery market is expected to reach $115 million by 2022, growing at a CAGR of 38.4% during the forecast period. The automotive industry is estimated to dominate the market throughout the analysis period. Geographically, Europe is expected to be the leading market in 2016, with a revenue contribution of around 38%.”
With ever-increasing energy demands all across the world, developing improved energy storage devices with reduced negative environmental impacts related to consumer based battery usage is a noble objective and one that Cheap Tubes definitely supports. Cheap Tubes hopes that this guide has helped you to know the current graphene battery research trends and inspired you to initiate graphene battery development.
References
- https://www.researchgate.net/publication/236835746_A_Fibrous_Hybrid_of_Graphene_and_Sulfur_Nanocrystals_for_High_Performance_ Lithium-Sulfur_Batteries
- Huang X., Xiaoying Q., Boey F. and Zhang H., Graphene based composites, Chem Soc. Rev., 2012, 41, 666-686
- Zhou G., Yin L., Wang D. and Cheng H., A fibrous hybrid of graphene and sulfur nanocrystals for high performance lithium-sulfur batteries, ACS Nano, 2013, 7(6)
- Cheng Q., Tang J., Zhang H., Graphene and carbon nanotube composite electrodes for supercapacitors with ultra-high energy density, Phys. Chem. Chem. Phys., 2011, 13, 17615-17624
- Peng Z., Xiang C., Yan Z., Natelson D., Graphene Nanoribbon and Nanostructured SnO2 Composite Anodes for Lithium Ion Batteries, ACS Nano, 2013, 7(7)
- Haegyeom K., Dong-Hwa S., Sung Wook None K., Kisuk K., Highly reversible Co3O4/graphene hybrid anode for lithium rechargeable batteries, Carbon, 2011, 49(1), 326-332
- https://www.researchgate.net/publication/236835746_A_Fibrous_Hybrid_of_Graphene_and_Sulfur_Nanocrystals_for_High_Performa nce_Lithium-Sulfur_Batteries
- http://www.graphenea.com
- http://www.businesswire.com/news/home/20161101006012/en/Global-Graphene-Battery-Market-Worth-USD-115
- http://www.mdpi.com/2079-4991/5/3/1481/htm
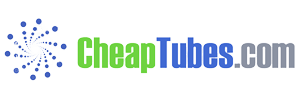
This information has been sourced, reviewed and adapted from materials provided by Cheap Tubes Inc.
For more information on this source, please visit Cheap Tubes Inc.