Many life science researchers will have to re-evaluate their preclinical workflows in light of developments of NIR-II or in vivo imaging in the second biological window, although this technology is in its earlier stages.
Due to autofluorescence, light scattering and absorption in living tissues, there is an inability to localize living signals in preclinical optical imaging. A signal that is at the surface but not deep in the organism can be localized by in vivo optical imaging.
Discussions between preclinical biologists and imaging physicists about their strong desire to be able to rapidly localize optical signals in vivo have thus far been unfruitful.
The questions from biologists about the process have been about whether or not optical imaging can be used to see luciferase cells or mCherry cancer cells in vivo.
The answer depends on many factors, including how many photons come out, how deep they are, the optical properties of organs and the temperature of the animal.
NIR-II in vivo imaging is able to image optical probes in real-time much more deeply in the organism and with much higher resolutions because it is not impacted in the same way by light propagation in living tissues.
NIR-I and NIR-II probes have been demonstrated to work well for this application in the field of SWIR imaging. There are many probes that have been used with the new imagining modality, though many remain to be validated.
The next steps of progress need to be taken by biologists. Biologists no longer need to accept ‘it depends’ as an answer from an optical imaging physicist when asking if it is possible to localize probes in vivo.
A Short History of Small Animal Optical Imaging
In the mid-1990s, David Beneron, together with Pamela and Chris Contag at Stanford University, originated optical imaging of small animals, now a widely-used technique. The scientists used a highly sensitive CCD camera to track optical signals in vivo by looking at bacterial infections in mice.
This early technology was cost-effective in comparison to MRI and PET imaging, which was one of the key drivers in its development. It was additionally a safe and non-invasive modality and had rapid output, high spatial resolution and had visualization capabilities.
Lab benchtops all over the world could make use of the in vivo imagers’ integrated cooled CCD camera configuration and desktop feel. Because everything was in vivo, it meant a smaller cohort size when doing toxicology studies and drug discovery.
There are now thousands of imaging systems used every day to monitor disease model progressions in laboratory models all over the world.
In vivo, optical imaging has its own disadvantages and advantages, the same as other imaging modalities. It has poor image sensitivity and resolution for signals more than a few millimeters deep.
However, for optical signals coming from the surface, it has great sensitivity, which allows for early monitoring and detection of small changes.
Developments were made in the mid-2000s to assist in the recovery of signals deep within the organism using modified configurations that used trans-illumination modes of excitation. This allowed the recovery of fluorescence signal depth. Additionally, the height of the animal for bioluminescent imaging was measured using a profilometer.
The first diffuse optical 3D tomography images in living systems were made using the newly developed imagers, which relied on algorithmic estimations. The success of this method would have circumvented the need for ex vivo biodistribution studies in preclinical testing, thereby further reducing cohort sizes.
Although the next generation imagers in this new configuration presented an option to generate 3D images, there were still challenges due to complications of light diffusing in living tissues.
For example, a 3D reconstruction is off tenfold1 if the estimation algorithms are off by 1 mm. Because of this, modern publications still only contain 2D images even when they have 3D imagers.
The fundamental issues of light propagation in living systems remain, despite the implementation of X-ray and CT as a way to help with localization at depths. Optical tomography in the visible wavelengths has physical limitations that are not alleviated completely by X b-ray and CT.
The NIR-II Window, a Sweet Spot for In Vivo Imaging
SWIR, or shortwave infrared, is another name for NIR-II, or second near-infrared spectral region, is defined most often as the wavelength range from 900 to 1700 nm.
It is still not fully clear exactly why in vivo imaging works so well in this spectral window, but there are a few certainties2: there are lower levels of absorption of light, scattering and autofluorescence in the NIR-II wavelengths of 1000 to 1700 nm and SWIR emitters are excited by NIR-I light which has better penetration.
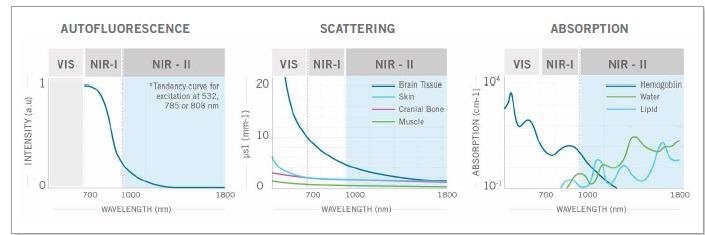
Figure 1. The weaker autofluorescence by the tissues in the NIR-II window contributes to enhancing the signal-to-background ratio. The reduced scattering and minimal absorption of emission signals by the tissues helps to improve spatial resolution and depth penetration, which can be up to 10x better. Image Credit: Photon etc
Preclinical Applications
It is very likely that NIR-II will bring lower cost high resolution imaging that is an unprecedented combination of fast and deep.
This will mean contact-free cardiography, vascular anatomy, visualizing tumors and other preclinical imaging techniques will be accessible to institutes without access to micro-MRI or micro-CT imaging systems, which are more costly.
Below are some examples of how this new modality will make some of the basic, preclinical applications possible.
Visualizing Blood Vessels and Mapping Blood Flows
In vivo real-time visualization is able to image blood flow and vascular anatomy, meaning that it has substantial potential for improving understanding of angiogenesis and circulatory system-related pathologies.
Magnetic resonance imaging (MRI) and micro-computed tomography (micro-CT), two current methods for assessing vascular structures), have unlimited penetration depth but complicate real-time imaging in small animals because of their long acquisition and post-processing times.
In a 2012 paper3, Hongje Dai’s group demonstrated that NIR-II imaging could be used to map venous and arterial blood flows beyond what ultrasound at lower velocities is able to do.
It may be possible for oxygenation and tumor hemodynamics to be modeled using such blood flow mapping. The capabilities of this method can be used for imaging brain response to stimuli or muscle motion, among other activity states; these activities are closely linked to perfusion.
The angiogenic effects of blood vessel growth modulation in animal models of peripheral artery disease, meaning both in diseased and healthy tissues, have been extensively studied using murine hindlimb models.
The ability to assess angiogenic therapies’ effectiveness and visualizing hindlimb ischemia are two uses for high resolution imaging of the blood vascular network.
Cancer research is also an important area when considering the uses of effective angiogenic modulation. An adequate blood supply is necessary for the growth and metastasis of a solid tumor with a diameter of over 1 mm.
NIR-II, with its economical price point, could provide the answer in the search for a simple and efficient method to monitor tumor angiogenesis for early detection and therapy research.
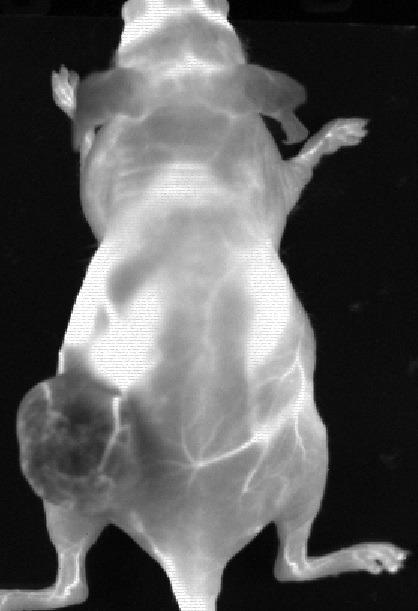
Figure 2. Data from an experiment mouse that had Ovarian tumour (SKOV-3) in the left flank following an IV injection of PbS QDots that have peak emission 1300 nm. The negative contrast in the tumour indicates a tumour barrier preventing contrast perfusion into the tumour. We also clearly see the blood vessels feeding the tumour. Image Credit: The Preclinical Imaging Laboratory of the National Research Center in Ottawa
Visualizing Tumours
Being able to see tumors in small animals earlier will also benefit early detection research. In tumor biology, fluorescence and bioluminescence imaging have the advantage of being sensitive and detecting small changes.
However, these techniques, in xenograft studies where the tumor is at the surface, have replaced the caliper.
Probes activated by lysosomal enzymes, receptor binding and passive targeting (such as targeting via lymph nodes or EPR effect) make it possible to visualize tumors with positive contrast.
Bawendi’s MIT group also imaged HER-2 amplified breast cancer BT474 cells brain parenchyma successfully suing IV administration of Licor’s IRDye800 conjugated with trastuzumab (shown in Figure 3).
![Targeted SWIR imaging in vivo with IRDye 800 CW of a nude mouse with a brain tumour from implanted human BT474 breast cancer cells.Three days after injecting IRDye 800 CW–trastuzumab conjugate, fluorescence from the labelled tumour was imaged noninvasively through skin and skull on a SWIR camera. [4]](https://www.azonano.com/images/Article_Images/ImageForArticle_5667_16152668743896526.jpg)
Figure 3. Targeted SWIR imaging in vivo with IRDye 800 CW of a nude mouse with a brain tumour from implanted human BT474 breast cancer cells.Three days after injecting IRDye 800 CW–trastuzumab conjugate, fluorescence from the labelled tumour was imaged noninvasively through skin and skull on a SWIR camera.4. Image Credit: Photon etc
Blood vessels surrounding the tumor were additionally shown by using a PEGylated version of the Licor dye.4
Contact-Free Measurements of Cardiography, Respiratory Rate and Intestinal Contractions
Contact-free respiratory rate measurements and cardiography can be used on both awake and anesthetized animals using the high speed imaging made available by the fast frame rates of InGaAs cameras.
This was demonstrated by Bawendi’s research group at MIT, who used a blood pool contrast agent-based micellar InAs-Cd-Se-CdS shortwave infrared quantum dots with high quantum yield to generate enough signal-to-noise ratio for mice (both awake and anesthetized) to show the deep influence of anesthesia5 on physiology.
No restraining devices were needed to take the contact-free measurements of awake mice. Photon etc. scientists, working with the Preclinical Imaging Laboratory of the National Research Center in Ottawa also recently monitored the heart and respiratory rates of an anesthetized mouse.
The mouse’s intestinal contractions were also measured by the study, the values of which were corroborated by scientific publications (seen in Figure 4).
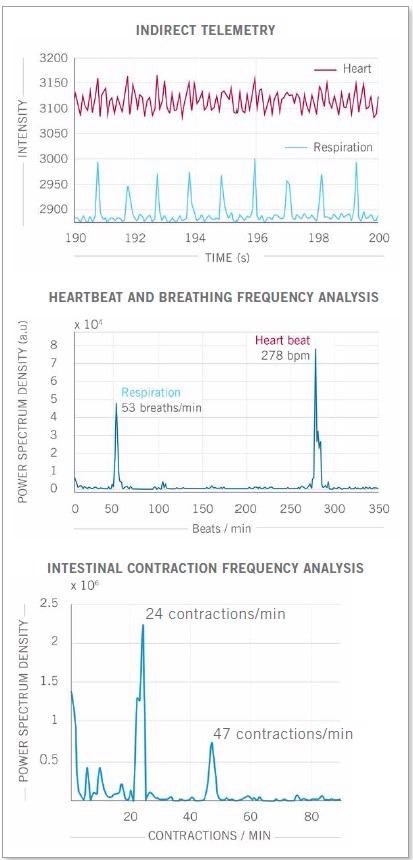
Figure 4. Real-time imaging data on heartbeat, respiratory rate and intestinal contractions obtained on Photon etc.’s IR VIVO reflect values found in the literature. Image Credit: Photon etc
Real-Time Monitoring of In Vivo Targets for Therapeutics Development
Real-time pharmacokinetic imaging of over a thousand targets simultaneously in a single mouse facilitated by NIR-II imaging could impact the development of drug discovery and targeted therapeutics on a fundamental level.
Figure 5 shows an example of a real-time kinetic curve at several locations on the mouse, obtained simultaneously and rapidly by new NIR-II imaging technologies that are designed to offer time profiling analysis for each region of interest (ROI) and pixel in a single click.
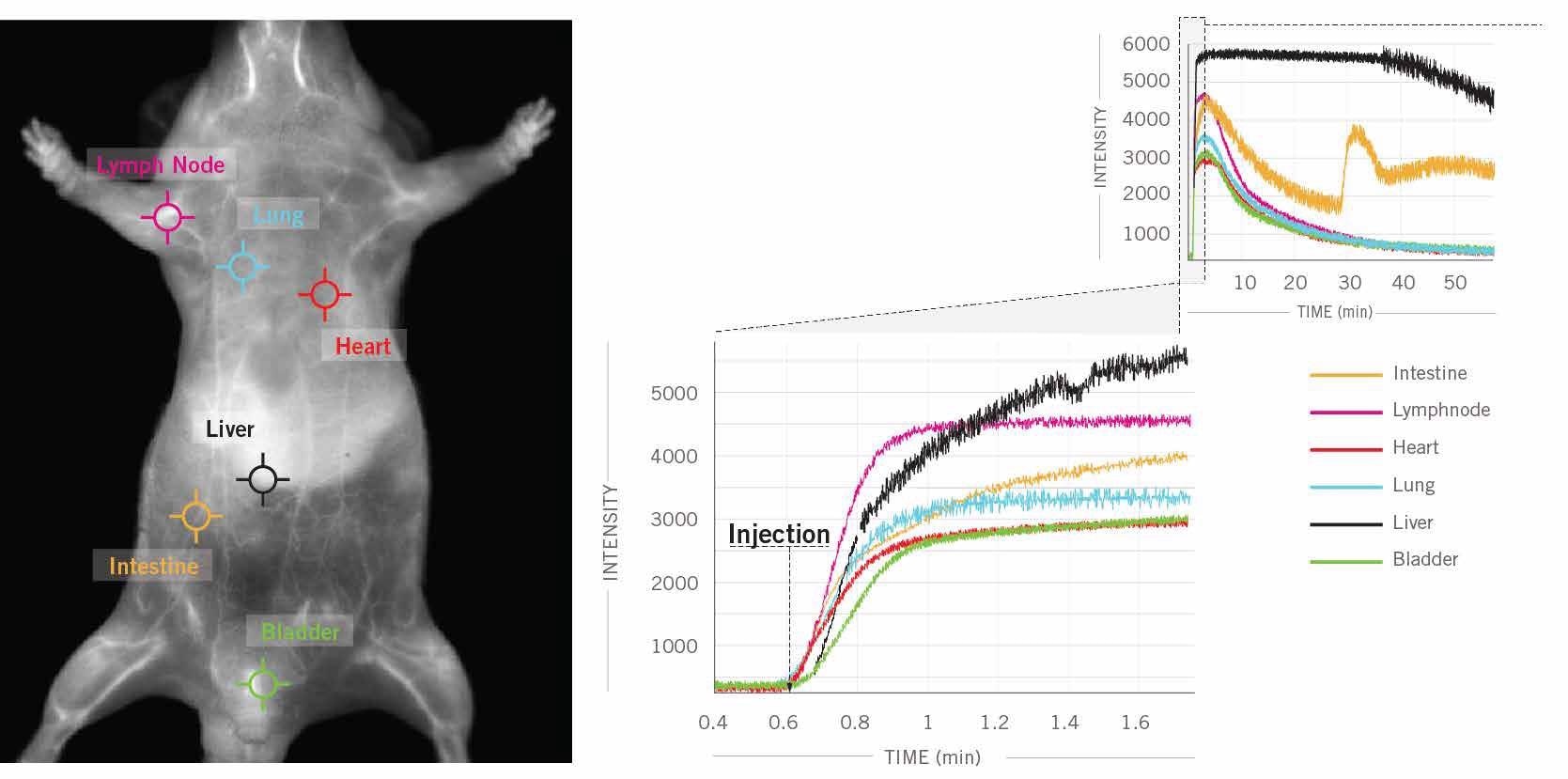
Figure 5. In this example, 6 clicks were used to obtain kinetic curves at 6 different locations (intestine, lymph nodes, heart, lung, liver, bladder) on the mouse’s body for a one hour scan following ICG injection in a male CD1 mouse. Image Credit: Photon etc
Major organs and tissues may be precisely delineated by using a time series of fluorescence imaging that has had principal component analysis (PCA) applied to it.
The kinetics of fluorescent intensity changes can be measured with one click spectrum extraction. This allows scientists to compare relative signal ratios between organs and to determine the elimination and accumulation of probes in selected organs or regions.
The information provided by this process can be used to obtain values from gastrointestinal transit rates and hepatobiliary elimination and can assist in understanding, in detail, how the probes are metabolized by the biological system.
The probe development community should be interested in being about to use a single mouse to acquire such a wealth of biodistribution data.
Probe development has historically used near-infrared imaging with anticancer agents conjugated to NIR dyes. By shifting detection technologies to NIR-II, these programs would be enhanced and would not have to rely on organ extraction to determine biodistribution. This can be seen in Figure 6.
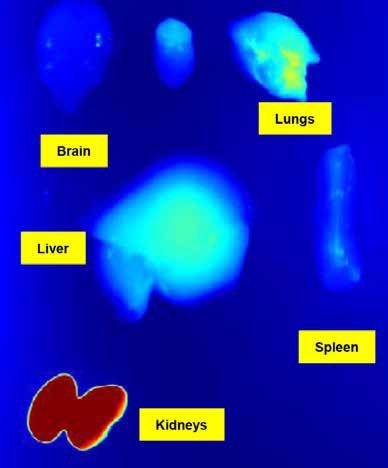
Figure 6. Studies ex vivo won’t be necessary anymore to get the biodistribution data in each organ. Image Credit: The Preclinical Imaging Laboratory of the National Research Center in Ottawa
Cohort sizes for preclinical studies can also be further reduced by using the same animal for multiple PK analyses. Through the use of NIR-II, researchers could use in vivo imaging earlier in the probe validation process, meaning NIR-II would have a much more fundamental role in compound screening.
Study design, especially as relating to preclinical testing probe validation (seen in Figure 7), will undoubtedly be affected by NIR-II in vivo imaging’s inclusion in preclinical workflow.
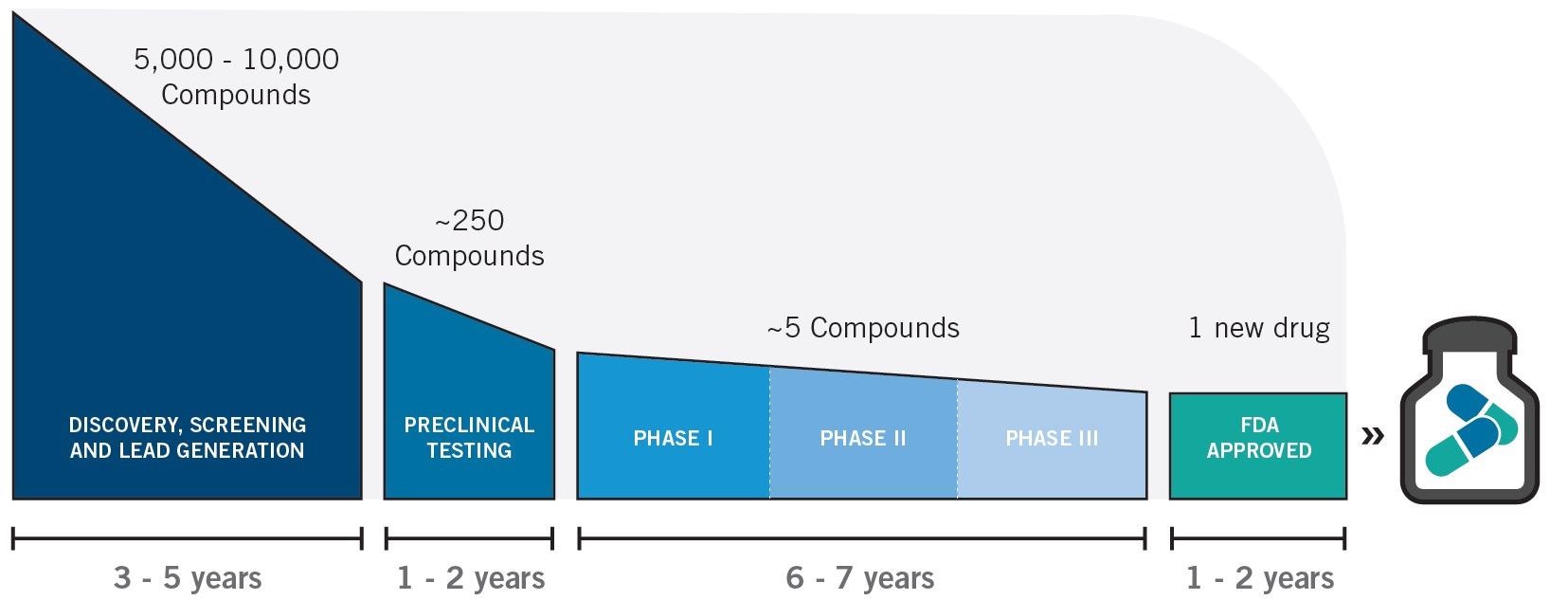
Figure 7. Therapeutics drug and development timeline. Image Credit: Photon etc
It is not possible to acquire a real-time determination of the proportion of probes accessing various regions of an organism using NIR-I in vivo imaging. Additionally, biodistribution data is often obtained via organ harvesting for ex vivo analyses.
However, it is possible to track minute changes in real-time and to examine very small concentrations of probes through various organism ROIs by using NIR-II in vivo imaging.
Metabolic Imaging
Metabolic information for the study of metabolic diseases can also be conveniently obtained used PK analyses via high speed NIR-II imaging.
The progression of metabolic diseases can be staged and monitored using different contrast agent techniques like standard ICG, the encapsulation of ICG in emulsions and SWIR Qd nanosomes by examining how hepatocyte metabolism and/or adipose tissue physiology impacts how time courses are modified.
Photon etc.’s study with ICG measured both hepatobiliary elimination and peristaltic movements of the intestine, as can be seen in Figure 8. This is likely to be the first time that the intestinal contractions of small animals were measured using in vivo imaging.
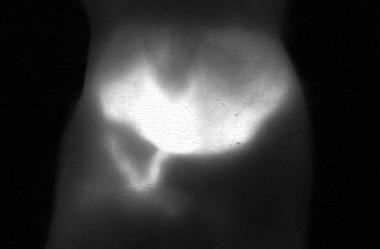
Figure 8. Picture of mouse liver 22 minutes after injection of ICG: the image is taken from a video that shows the metabolism and hepatobiliary elimination of ICG into the intestine as well as intestinal contractions. Image Credit: Photon etc
There are limitations of breathing artifacts in modalities such as micro-CT imaging, which is possibly why there are fewer reported studies of gastrointestinal systems.
In vivo imaging is a much more straightforward method for imaging the intestine, which could make this kind of work more accessible to scientists researching diseases such as irritable bowel syndrome (IBS) or Crohn’s disease.
Scientists were able to detect lipid accumulation of NAFLD (non-alcoholic fatty liver disease) in a different metabolic study by Photon etc., that used their IR Vivo imaging system.
This study showed that after even reverting to a normal diet, the elevated lipid quantities in hepatic macrophages from a high fat diet persisted.
This study indicated the potential for accelerating preclinical research for staging and monitoring fatty liver disease6 progression through researchers being able to dynamically monitor endolysosomal lipid accumulation in vivo over time scales ranging from weeks to minutes.
Translational Research
Preclinical imaging in the SWIR wavelengths has the strong advantage of having translational potential. The same model of InGaAs camera used by many labs for preclinical research is the same as was recently used in the first in-human clinical study.
This clinical study also provided an enhanced tumor-detection rate, a higher tumor-to-normal-tissue signal ratio and higher tumor-detection sensitivity by using NIR-II instead of NIR-I, confirming its advantages.
Working in the NIR-II window7 also has reduced background and improved depth penetration, similar to the preclinical story.
Most other imaging modalities do not have the ability to translate directly into the clinic. As an example, a clinical computer tomography and a micro-CT system use different detection technology.
The imaging probes of NIR-II are also pervaded by translational research. The discovery that standard NIR probes are also SWIR emitters has been one of the major breakthroughs in imaging research of recent years.
In a paper published in PNAS, Carr et al. suggested that the perceived need for probes with peak emission in NIR-II and the lack of SWIR imaging hardware is behind preclinical research only recently integrating NIR-II.
Silicone-based spectrometers did not have the quantum efficiency required in SWIR wavelengths for the detection of long NIR-II emission tails of standard NIR-I dyes like Licor’s IRDye 800CW or ICG, which means that they did not provide an accurate picture of emission spectra.
The paper also argued that ICG, a NIR-I dye (FDA approved in 1957), is significantly brighter than NIR-II IR-E1050 dye8, which is commercially available, which is the current in vivo benchmark.
Testing FDA-approved probes and working to re-purpose some pre-existing NIR-I probes to increase their tail emissions into the NIR-II may be an optimal strategy for translational research.
The use of NIR-I dyes in clinical and translational SWIR imaging research has been predicted by Dr. Xiaoyuan Chen and colleagues from the National Institutes of Health (NIH).
There are two reasons for this. The first is that investigational new drug (IND) applications have already been completed for these fluorophores, and the other is that NIR-I dyes have an off-peak NIR-II emission.
This means that these dyes do not need to follow the same validation process as newly-developed NIR-II dyes to achieve clinically significant emissions in the surgery room. It is likely that surgical outcomes2 will also be improved by incorporating InGaAs detectors into current clinical NIR-Imaging hardware.
Continued efforts for the optimizations of quantum yields for dyes with peak emissions in the NIR-II is also an important part of this translational research, as well as working to improve NIR-II emissions of NIR-I dyes with long emission tails.
Optical imaging modality cannot access beyond the center depth and so will not compete with MRI and CT in clinical settings.
This demonstrates how it is important to combine multimodal approaches with NIR-II translational research programs such as NIR-I dye with a long emission tail conjugated to the development of MRI/PET/Ultrasound probes.
The Abundance of Contrast Agents for NIR-II Imaging
Existing research programs examining the development of probes with peak emissions in SWIR wavelengths can be complemented by the discovery that long-tail emissions of NIR probes can be used for NIR-II imaging.
Figure 9 shows NIR-II contrast agents that have been developed in recent years by research groups around the world, including aggregation-induced emission (AIE) probes9, semiconducting polymer-based nanoparticles (SPNPs), down conversion rare-earth nanoparticles, nanoparticle alloys, graphene dots, semiconductor quantum dots, single-walled carbon nanotubes and small molecule-based dyes.
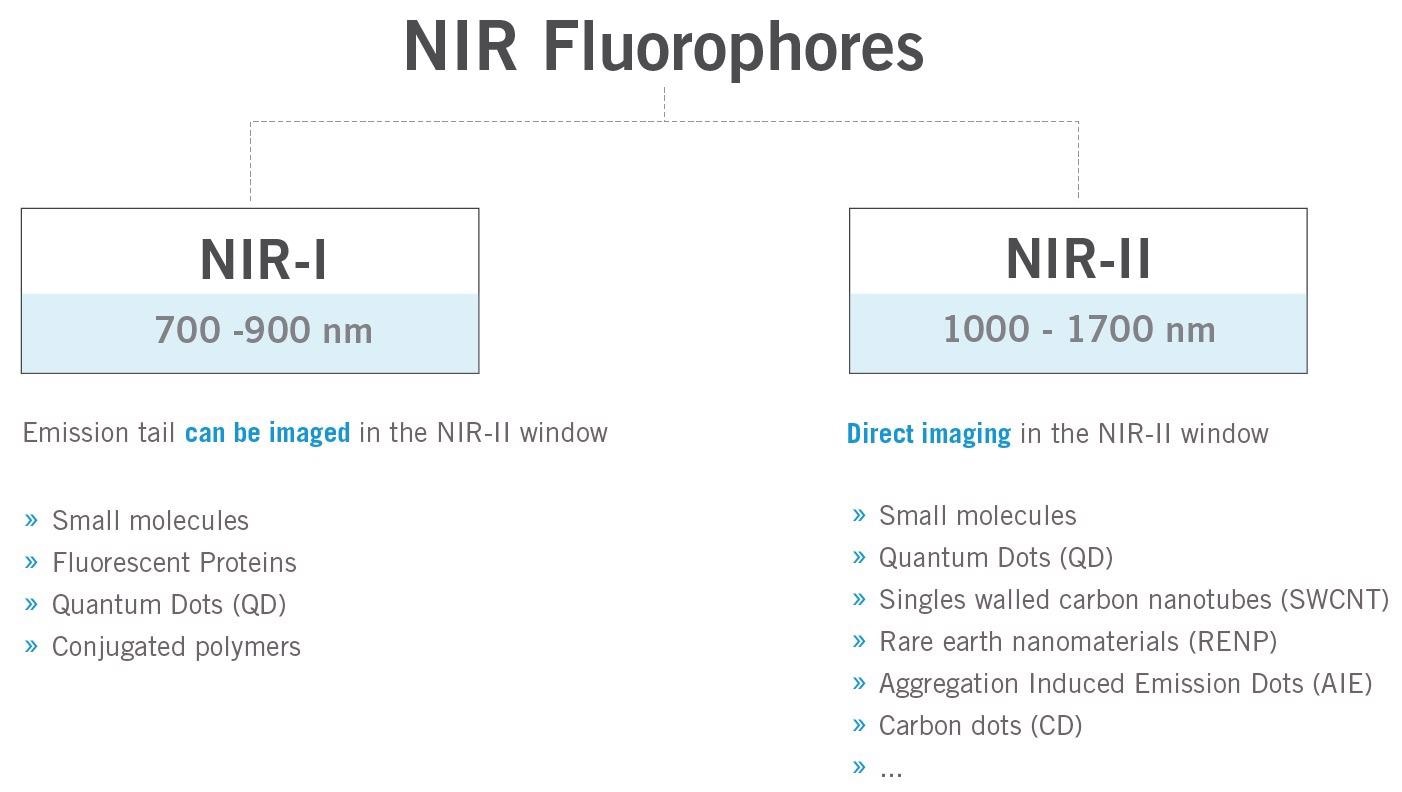
Figure 9. Brief overview of the typical fluorophores for in vivo fluorescence imaging in the near-infrared region. Image Credit: Photon etc
The superior penetration depth at these wavelengths is taken advantage of by these contrast agents. The contrast agents are likely to be able to generate new tools because of their unique properties, including disease monitoring and diagnosis10, theranostics, treatment efficacy assessment and surgery guidance.
An example of this is the detection of microRNA or lipid content allowed by carbon nanotube sensors. Fan content could be mapped in real-time thanks to the shifting of the emission wavelength of the carbon nanotubes (dependent on the cellular environment).
It may be possible to image deeper into the organism by pushing peak emissions further into the NIR-II, allowing for greater precision in the detection of tumor lysosomal activity and the performance of multispectral imaging with non-overlapping emissions spectra because of narrow emission bands of materials like single-walled carbon nanotubes and quantum dots.
References
- Courtesy of MediLumine. Values obtained from measurements with the PRISM system.
- Zhu, S. et al. Near-Infrared-II (NIR-II) Bioimaging via Off-Peak NIR-I Fluorescence Emission. Theranostics 8, 4141–4151 (2018).
- Hong, G. et al. Multifunctional in vivo vascular imaging using near-infrared II fluorescence. Nature Medicine 18, 1841–1846 (2012).
- Carr, J. A. et al. Shortwave infrared fluorescence imaging with the clinically approved near-infrared dye indocyanine green. Proc. Natl. Acad. Sci. U. S. A. 115, 4465–4470 (2018).
- Bruns, O. T. et al. Next-generation in vivo optical imaging with short-wave infrared quantum dots. Nat. Biomed. Eng. 1, (2017).
- Galassi, T. V. et al. An optical nanoreporter of endolysosomal lipid accumulation reveals enduring effects of diet on hepatic macrophages in vivo. Sci. Transl. Med. 10, eaar2680 (2018)
- Hu, Z. et al. First-in-human liver-tumour surgery guided by multispectral fluorescence imaging in the visible and near-infrared-I/II windows. Nat. Biomed. Eng. (2019).
- Starosolski, Z. et al. Indocyanine green fluorescence in second near-infrared (NIR-II) window. PLoS One 12, 1–14 (2017).
- Cao, J. et al. Recent Progress in NIR-II Contrast Agent for Biological Imaging. Front. Bioeng. Biotechnol. 7, 1–21 (2020).
- He, S. et al. Crucial breakthrough of second near-infrared biological window fluorophores: design and synthesis toward multimodal imaging and theranostics. Chem. Soc. Rev. 47, 4258-4278 (2018).
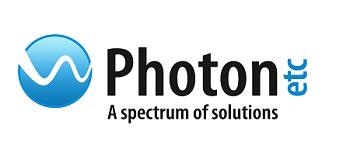
This information has been sourced, reviewed and adapted from materials provided by Photon etc.
For more information on this source, please visit Photon etc.