Si has emerged as a possible candidate for next-generation Li-ion battery anodes due to its better theoretical gravimetric capacity than graphite. Additionally, Si in nanowire (NW) form is better able to handle the unfavorable impacts of volume expansion and contraction during cycling, as well as the resulting loss of current collector contact.
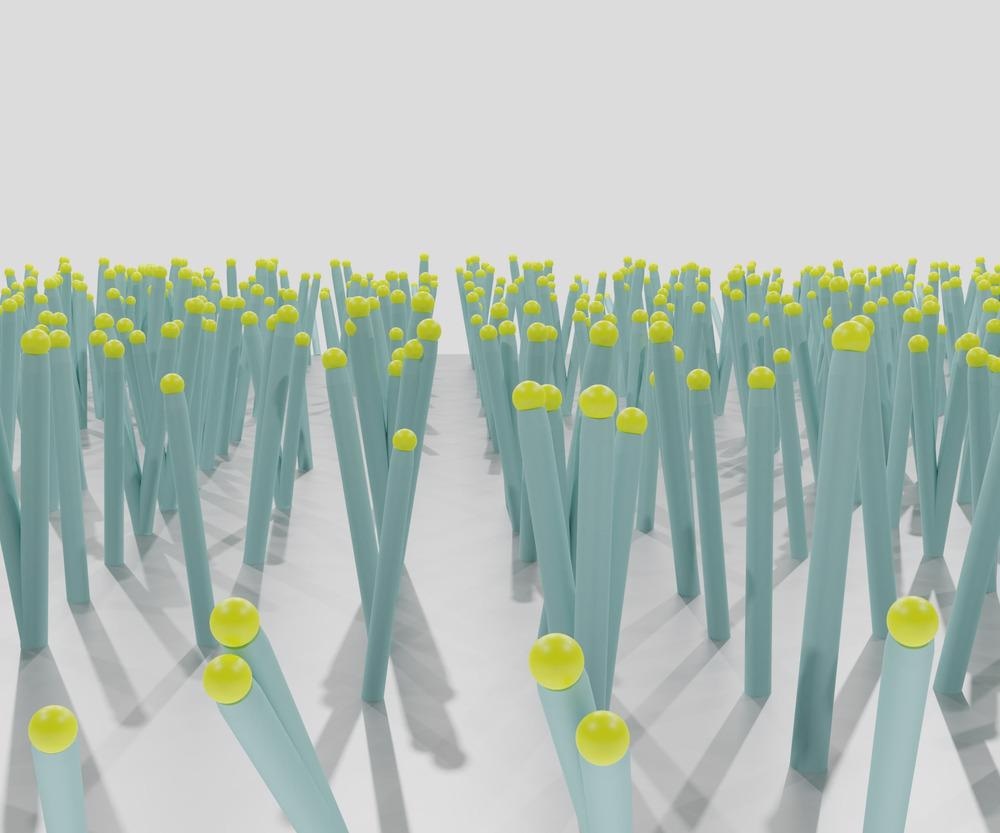
Image Credit: Love Employee/Shutterstock.com
SiNWs may also be generated directly from the electrode current collector, eliminating the requirement for inert electrode materials. However, there are still issues with Si NW manufacture and scalability, particularly when using complicated and expensive chemical vapor deposition (CVD) equipment.
Another barrier to wide-scale use of directly-grown NW anodes is the low mass loadings (usually <0.5 mg/cm2) and associated areal capacities obtainable by traditional synthetic techniques. Due to their enhanced nucleation sites for Si NW development, high surface area (HSA) current collectors provide a promising solution to this problem, allowing for greater mass loadings.
Si NWs grown on flexible HSA carbon substrates had previously been shown to be more suitable for wearable electronics or flexible devices, with the carbon cloth (CC) having the extra benefit of storing additional charge.
The creation of a woven Si/C fabric with weight loadings of 13.75 mg/cm2 and areal capacities of 14.3 mAh/cm2 is a more recent development on flexible Si-based anodes. Microscrolled, carbon-coated Si nanoparticles were fixed on conductive carbon nanotubes and then restricted in cellulose carbon rolls as an alternative strategy to generate flexible Si-based anodes.
Although good cycling stability over 800 cycles was observed, production of the active substance required long processing times (>48 hours) and high temperatures (700 °C).
For HSA CC substrates, researchers describe a Si NW synthesis technique in a new study published in Material Today Energy The method combines the advantages of the previously described growing system, which is a simple glassware-based system that uses liquid Si precursors at low temperatures (460 °C) and ambient pressures, with solvent-free/CVD conditions that are ideal for Si NW growth on CC.
When compared to NWs grown on planar substrates, the CC substrate guarantees that the anodes are flexible and allow for a larger mass loading. Higher areal capacities (2.0 mAh/cm2 on CC versus 0.3 mAh/cm2 on SS), as well as consistent cycling performance and high rate capabilities, result from this.
Methodology
Fluorochem provided phenylsilane (PS, 97%) and kept it in an Ar-filled glovebox. To enhance the surface area, stainless steel (SS, 316) foil with a thickness of 0.1 mm was roughened with sandpaper. Reactions were carried out in a Pyrex 100 mL round-bottomed flask with a long neck. Before being placed in the flask, the growth substrates were placed in a custom-made holder. A water condenser connected the flask to a Schlenk line arrangement.
Two electrode coin cell (CR2032) type cells were assembled in an Ar-filled glovebox to test electrochemical performance. Si NWs on an SS (or CC) current collector, Li foil as the counter and reference electrode, and a Celgard separator made up the cells.
On a Hitachi SU-70 system based between 5 and 20 kV, a scanning electron microscopy (SEM) investigation was done. The NWs were sonicated from the growing substrate before being drop cast onto a lacy carbon TEM grid for transmission electron microscopy (TEM) examination.
Results
Figures 1a and b show the Si NW synthetic method, which is a modification of the previously disclosed SVG NW growing procedure. In the reaction chamber, the technique allows for huge growth substrates (Figure 1c). CC is also very flexible, with no visible Si NW delamination when twisted or bent (Figure 1d).
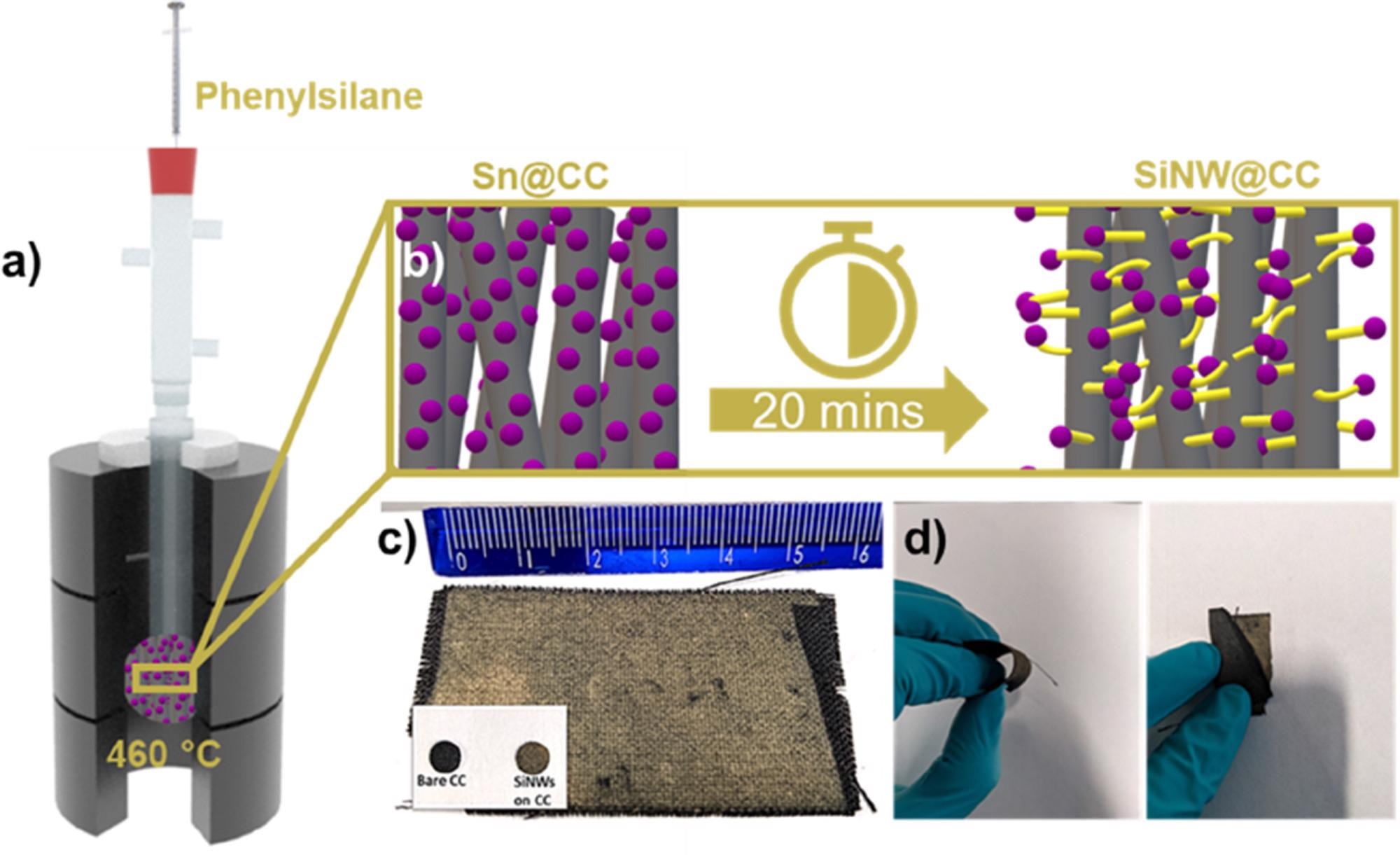
Figure 1. a) Schematic illustration representing glassware based CVD growth of Si NWs. (b) Schematic description of Si NW growth on CC. c) Optical photograph showing SiNW (matt yellow) coverage on CC (black) with bare CC anode and SiNW@CC anode (inset). d) Images showing the flexible nature of the SiNW@CC substrates. Image Credit: Storan, et al., 2022
CC was used as an HSA conductive current collector substrate with the optimal Si NW growth conditions (460 °C, 20 minutes) (Figure 2a-c). The fibrous structure of CC is shown via SEM examination (Figure 2a). With Sn seeds, SEM exhibited dense coverage of all fibers on the substrate (Figure 2b). The high density of Si NWs formed on the CC fibers was found by post-synthesis SEM examination (Figure 2c).
Si NWs are so densely packed that the individual carbon fibers’ surfaces are no longer visible. A seeded NW was discovered using TEM and STEM analysis (Figure 2d), with EDS mapping revealing a Sn (purple) seed with a Si (yellow) body (Figure 2e).
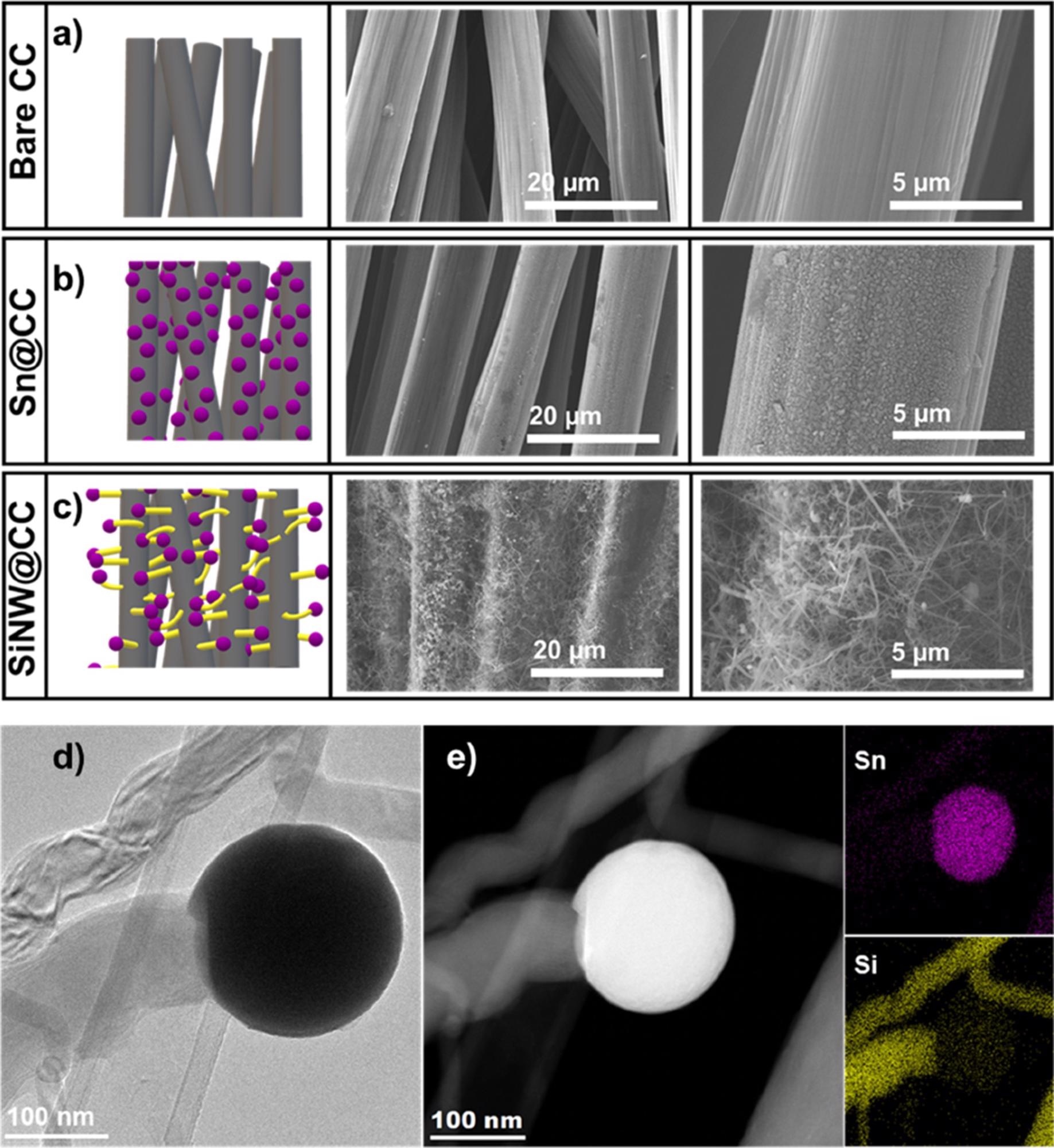
Figure 2. Schematic and SEM images of a) bare CC, b) Sn coated CC and c) SiNW coated CC. d) TEM and e) STEM image of a Sn seeded SiNW with corresponding Sn and Si EDS elemental maps. Image Credit: Storan, et al., 2022
In a 1 M LiPF6 in EC/DEC + 10 wt.% FEC at a current density of 200 mA/g, 1.1 mg Si/cm2 anodes were cycled in the voltage range of 0.01-1 V vs Li/Li+ (Figure 3a). The voltage profile study clearly shows negligible capacity decline after 200 cycles, demonstrating this high capacity retention (Figure 3b).
Figure 3c shows cyclic voltammograms (CVs) of bare CC and Si NW coated CC (SiNW@CC), which revealed that the SiNW@CC CV had more peaks than the bare CC-CV. Figure 3d shows that at 200 mA/g, areal capacities of 2.0 mAh/cm2 were attained in a rate capability test (RCT).
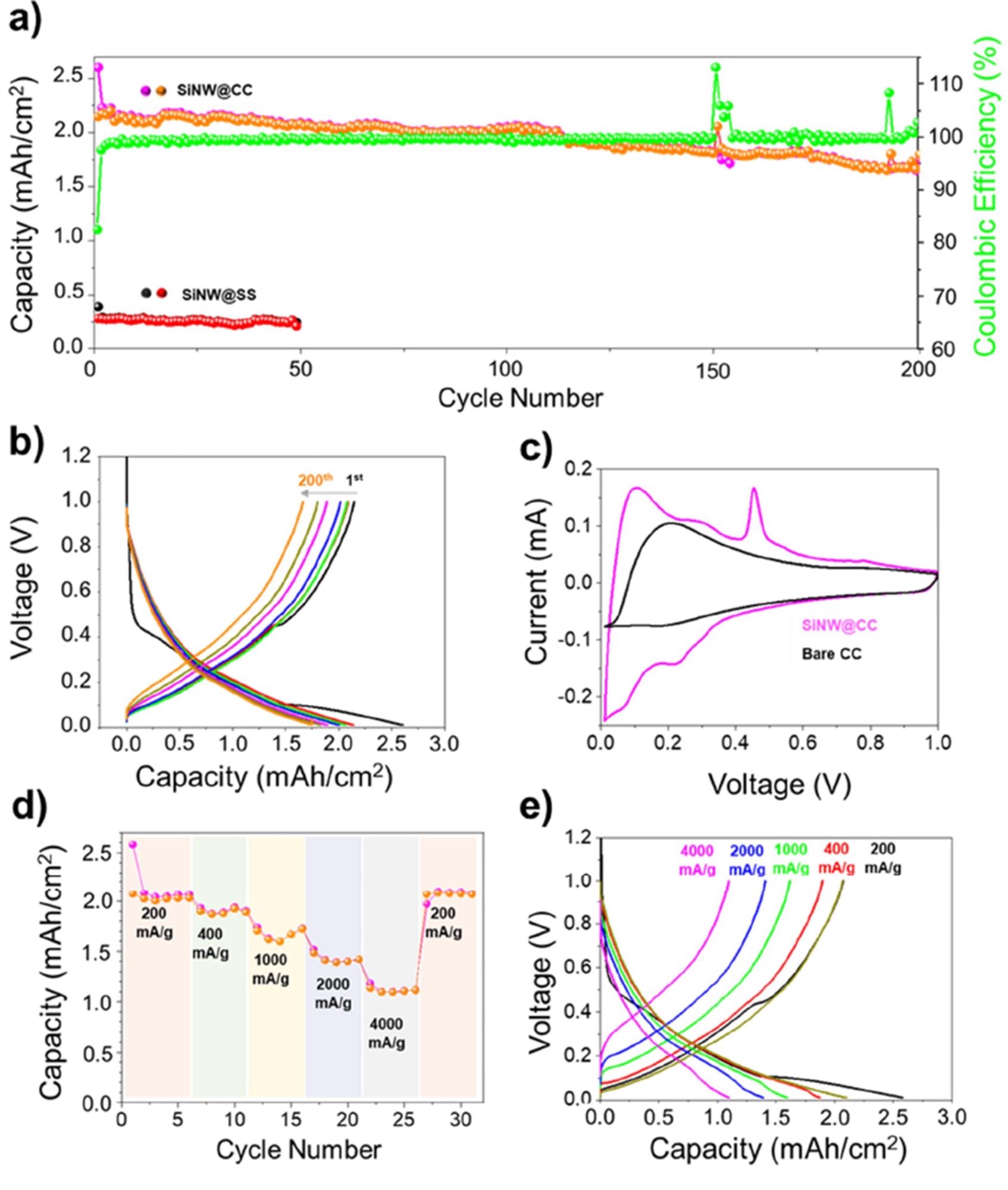
Figure 3. a) Columbic efficiency, charge and discharge areal capacities of SiNW@CC anode cycled at 200 mA/g, showing stable cycling performance and high Columbic efficiency. b) Voltage profile of SiNW@CC showing excellent capacity retention after 200 cycles. c) CV plots of bare CC (black) and SiNW@CC (purple) scanned at 0.025 mV s-1 between 0.01-1 V d) Rate capability testing of SiNW@CC anode showing the relationship between current density and achievable areal capacity. e) Voltage profiles of rate capability testing of SiNW@CC showing the decreasing capacity with increasing current density with good recovery when current density is returned to 200 mA/g. Image Credit: Storan, et al., 2022
After the first and 50th cycles of the SiNW@CC-based battery, electrochemical impedance spectroscopy (EIS) study was performed (Figure 4a). After the first and 50th cycles, the corresponding RS stayed the same, whereas RSEI reduced from 64.8 Ohm to 31.1 Ohm and Rct decreased from 198.10 Ohm to 110.1 Ohm, respectively (Figure 4b).
The production of porous interconnected and mesh-like Si was also confirmed by post-mortem SEM examination (Figure 4c, d).
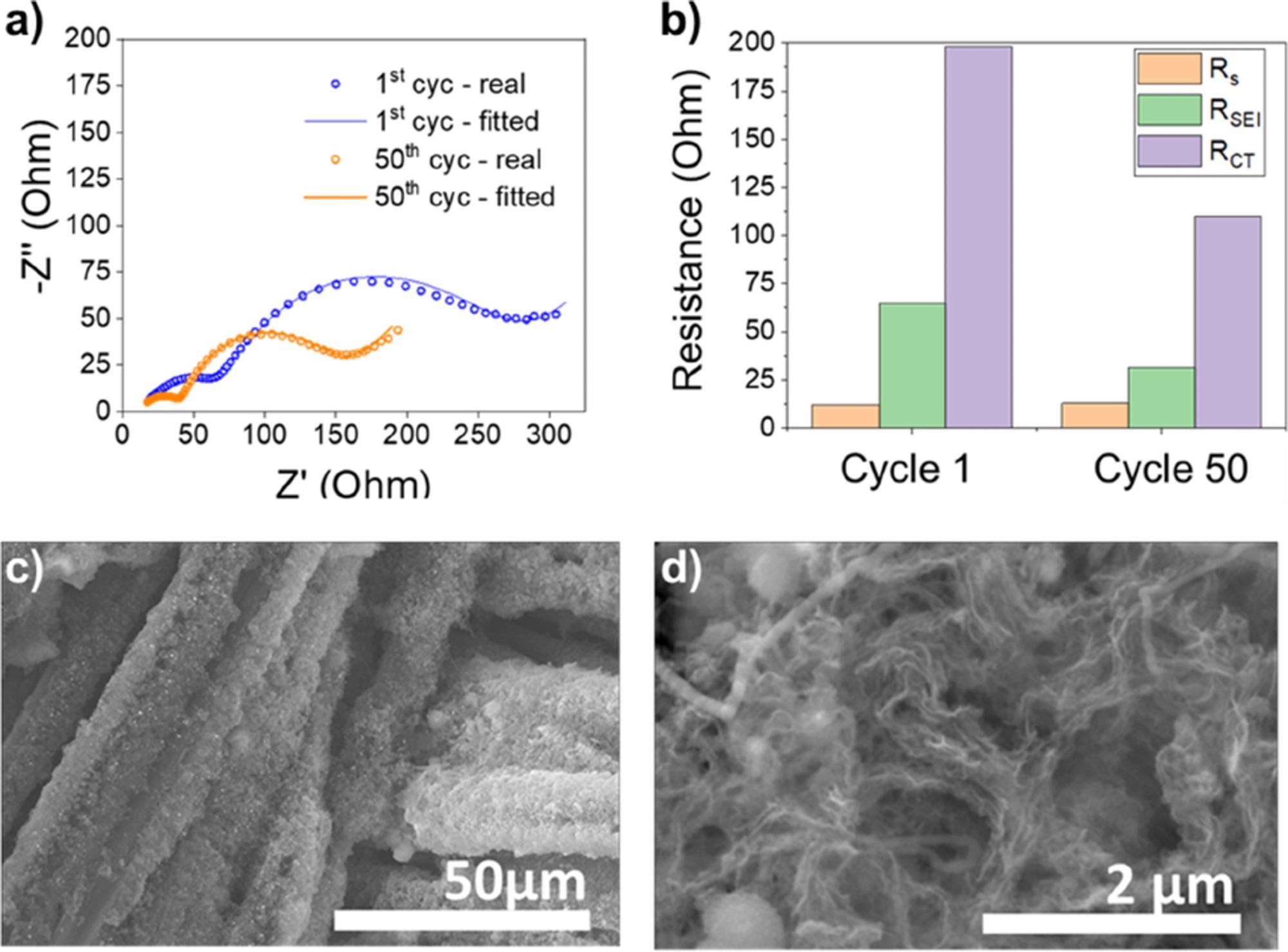
Figure 4. a) Nyquest plot of SiNW@CC cell after 1 cycle and 50 cycles. b) Bar chart revealing the difference in RSEI and RCT after the 1st and 50th cycles. c) And d) SEM images showing the porous amorphous Si ligament-like structure after 50 cycles. Image Credit: Storan, et al., 2022
The mesh exhibits good adhesion to the CC substrate, as evidenced by the fact that it is still adhering to it after 50 cycles with no symptoms of delamination from the CC current collector. The evidence of porosity network creation during cycling is further supported by post-mortem TEM examination.
Conclusion
Finally, researchers have shown that SiNW@CC can develop to be used as Li-ion battery anodes. In comparison to existing state-of-the-art CVD technologies, which employ greater temperatures, lower pressures, and longer reaction times, the growth conditions are appealing. Si NWs grown on CC have excellent charge and discharge capacities (>2mAh/cm2), excellent rate capability performance, and steady cycling capacity retention of 80% after 200 cycles.
This study demonstrated a practical approach for the quick and easy synthesis of Si NWs on CC for Li-ion battery applications.
Journal Reference:
Storan, D., Ahad, S. A., Forde, R., Kilian, S., Adegoke, T. E., Kennedy, T., Geaney, H. and Ryan, K. M. (2022) Silicon Nanowire Growth on Carbon Cloth for Flexible Li-ion Battery Anodes. Materials Today Energy. Available Online: https://www.sciencedirect.com/science/article/pii/S2468606922000880.
References and Further Reading
- Zhu, J., et al. (2016) Atomic-Scale Control of Silicon Expansion Space as Ultrastable Battery Anodes. ACS Nano, 10(9), pp. 8243–8251. doi.org/10.1021/acsnano.6b04522.
- Han, M., et al. (2020) Growth of flexible and porous surface layers of vertical graphene sheets for accommodating huge volume change of silicon in lithium-ion battery anodes. Materials Today Energy, 17, p. 100445. doi.org/10.1016/j.mtener.2020.100445.
- Wang, T., et al. (2017) Large-scale production of silicon nanoparticles@graphene embedded in nanotubes as ultra-robust battery anodes. Journal of Materials Chemistry A, 5, pp. 4809–4817. doi.org/10.1039/C6TA10631E.
- Zuo, X., et al. (2017) Silicon based lithium-ion battery anodes: A chronicle perspective review. Nano Energy, 31, pp. 113–143. doi.org/10.1016/j.nanoen.2016.11.013.
- Stokes, K., et al. (2018) Axial Si–Ge Heterostructure Nanowires as Lithium-Ion Battery Anodes. Nano Letters, 18(9), pp. 5569–5575. doi.org/10.1021/acs.nanolett.8b01988.
- Chan, C. K., et al. (2007) High-performance lithium battery anodes using silicon nanowires. Nature Nanotechnology, 3, pp. 31–35. doi.org/10.1038/nnano.2007.411.
- Stokes, K., et al. (2016) Direct Synthesis of Alloyed Si1–xGex Nanowires for Performance-Tunable Lithium Ion Battery Anodes. ACS Nano, 11(10), pp. 10088–10096. doi.org/10.1021/acsnano.7b04523.
- Chan, C. K., et al. (2008) High-performance lithium battery anodes using silicon nanowires. Nature Nanotechnology, 3, pp. 31–35. doi.org/10.1038/nnano.2007.411.
- Aminu, I. S., et al. (2020) A Copper Silicide Nanofoam Current Collector for Directly Grown Si Nanowire Networks and their Application as Lithium-Ion Anodes. Advanced Functional Materials, 30(38), p. 2003278. doi.org/10.1002/adfm.202003278.
- Stokes, K., et al. (2020) Influence of Carbonate-Based Additives on the Electrochemical Performance of Si NW Anodes Cycled in an Ionic Liquid Electrolyte. Nano Letters, 20(10), pp. 7011–7019. doi.org/10.1021/acs.nanolett.0c01774.
- Cui, L. -F., et al. (2009) Crystalline-Amorphous Core−Shell Silicon Nanowires for High Capacity and High Current Battery Electrodes. Nano Letters, 9(1), pp. 491–495. doi.org/10.1021/nl8036323.
- Collins, G. A., et al. (2021) A Nanowire Nest Structure Comprising Copper Silicide and Silicon Nanowires for Lithium-Ion Battery Anodes with High Areal Loading. Nano-Micro Small, 17(34), p. 2102333. doi.org/10.1002/smll.202102333.
- Wang, X., et al. (2017) Carbon-Coated Silicon Nanowires on Carbon Fabric as Self-Supported Electrodes for Flexible Lithium-Ion Batteries. ACS Applied Materials & Interfaces, 9(11), pp. 9551–9558. doi.org/10.1021/acsami.6b12080.
- Chang, C. -B., et al. (2021) Solution-Grown Phosphorus-Hyperdoped Silicon Nanowires/Carbon Nanotube Bilayer Fabric as a High-Performance Lithium-Ion Battery Anode. ACS Applied Energy Materials, 4(4), pp. 3160–3168. doi.org/10.1021/acsaem.0c02932.
- He, S -Y & Li, C -C (2022) Advantages of using carbon fabric over Cu foil as conductive matrix for anodes of micro- and nano-sized Si. Materials Research Bulletin, 148, p. 111690. doi.org/10.1016/j.materresbull.2021.111690.
- Shao, F., et al. (2021) Binder-Free, Flexible, and Self-Standing Non-Woven Fabric Anodes Based on Graphene/Si Hybrid Fibers for High-Performance Li-Ion Batteries. ACS Appllied Materials Interfaces, 13(23), pp. 27270–27277. doi.org/10.1021/acsami.1c04277.
- Wang, H., et al. (2020) A binder-free high silicon content flexible anode for Li-ion batteries. Energy Environment Science, 13, pp. 848–858. doi.org/10.1039/C9EE02615K.
- Zhu, R., et al. (2021) Silicon in Hollow Carbon Nanospheres Assembled Microspheres Cross-linked with N-doped Carbon Fibers toward a Binder Free, High Performance, and Flexible Anode for Lithium-Ion Batteries. Advanced Functional Materials, 31(33), p. 2101487. doi.org/10.1002/adfm.202101487.
- Geaney, H., et al. (2013) Solution phase synthesis of silicon and germanium nanowires. Journal of Materials Chemistry C, 1, pp. 4996–5007. doi.org/10.1039/C3TC31123F.
- Mullane, E., et al. (2013) Synthesis of Tin Catalyzed Silicon and Germanium Nanowires in a Solvent–Vapor System and Optimization of the Seed/Nanowire Interface for Dual Lithium Cycling. Chemistry of Materials, 25(9), pp. 1816–1822. doi.org/10.1021/cm400367v.
- Flynn, G., et al. (2018) Low temperature solution synthesis of silicon, germanium and Si–Ge axial heterostructures in nanorod and nanowire form. Chemical Communication, 54, pp. 5728–5731. doi.org/10.1039/C8CC03075H.
- Geaney, H., et al. (2012) High Density Growth of Indium seeded Silicon Nanowires in the Vapor phase of a High Boiling Point Solvent. Chemistry of Materials, 24(11), pp. 2204–2210. doi.org/10.1021/cm301023j.
- Zhao, Y & Guo, J (2020) Development of flexible Li-ion batteries for flexible electronics. InfoMat, 2(5), pp. 866–878. doi.org/10.1002/inf2.12117.
- Zhou, G., et al. (2014) Progress in flexible lithium batteries and future prospects. Energy Environmental Science, 7, pp. 1307–1338. doi.org/10.1039/C3EE43182G.
- Cha, H., et al. (2018) Issues and Challenges Facing Flexible Lithium-Ion Batteries for Practical Application. Nano-Micro Small, 14(43), p. 1702989. doi.org/10.1002/smll.201702989.
- Wagner, R S & Ellis, W C (1964) Vapor‐Liquid‐Solid Mechanism of Single Crystal Growth. Applied Physics Letters, 4, p. 89. doi.org/10.1063/1.1753975.
- Wang, H., et al. (2013) Atomistics of vapour–liquid–solid nanowire growth. Nature Communications, 4, p. 1956. doi.org/10.1038/ncomms2956.
- Kim, S., et al. (2017) Designing Morphology in Epitaxial Silicon Nanowires: The Role of Gold, Surface Chemistry, and Phosphorus Doping. ACS Nano, 11(5), pp. 4453–4462. doi.org/10.1021/acsnano.7b00457.
- Schmidt, V., et al. (2010) Growth, Thermodynamics, and Electrical Properties of Silicon Nanowires. Chemical Revies, 110(1), pp. 361–388. doi.org/10.1021/cr900141g.
- Tuan, H -Y & Korgel, B A (2008) Importance of Solvent-Mediated Phenylsilane Decompositon Kinetics for High-Yield Solution-Phase Silicon Nanowire Synthesis. Chemistry of Materials, 20(4), pp. 1239–1241. doi.org/10.1021/cm7033068.
- Hogness, T. R., et al. (1936) The Thermal Decomposition of Silane. Journal of the American Chemical Society, 58(1), pp. 108–112. doi.org/10.1021/ja01292a036.
- Coutant, R W & & Levy, A (1969) A kinetic study of the thermal decomposition of selected cyclohexyl and phenylsilanes; In: BATTELLE MEMORIAL INST COLUMBUS OH COLUMBUS, United States. Available at: https://apps.dtic.mil/sti/citations/AD0701730.
- Harris, J. T., et al. (2010) Hydrogenated Amorphous Silicon (a-Si:H) Colloids. Chemistry of Materials, 22(23), pp. 6378–6383. doi.org/10.1021/cm102486w.
- Pell, L. E., et al. (2004) Synthesis of Amorphous Silicon Colloids by Trisilane Thermolysis in High Temperature Supercritical Solvents. Langmuir, 20(16), pp. 6546–6548. doi.org/10.1021/la048671o.
- Etacheri, V., et al. (2012) Effect of Fluoroethylene Carbonate (FEC) on the Performance and Surface Chemistry of Si-Nanowire Li-Ion Battery Anodes. Langmuir, 28(1), pp. 965–976. doi.org/10.1021/la203712s.
- Chockla, A. M., et al. (2012) Tin-Seeded Silicon Nanowires for High Capacity Li-Ion Batteries. Chemistry of Materials, 24(19), pp. 3738–3745. doi.org/10.1021/cm301968b.
- Bogart, T. D., et al. (2014) Enhancing the lithiation rate of silicon nanowires by the inclusion of tin. RSC Advances, 4, pp. 42022–42028. doi.org/10.1039/C4RA07418A.
- Yoon, T., et al. (2015) Capacity Fading Mechanisms of Silicon Nanoparticle Negative Electrodes for Lithium Ion Batteries. Journal of The Electrochemical Society, 162(12), pp. A2325–A2330. doi.org/10.1149/2.0731512jes.
- Kennedy, T., et al. (2017) Understanding the influence of electrolyte additives on the electrochemical performance and morphology evolution of silicon nanowire based lithium-ion battery anodes. Journal of Power Sources, 359, pp. 601–610. doi.org/10.1016/j.jpowsour.2017.05.093.
- Jeong, Y. K., et al. (2020) Microclusters of Kinked Silicon Nanowires Synthesized by a Recyclable Iodide Process for High-Performance Lithium-Ion Battery Anodes. Advanced Energy Materials, 10(41), p. 2002108. doi.org/10.1002/aenm.202002108.
- Ruffo, R., et al. (2009) Impedance Analysis of Silicon Nanowire Lithium Ion Battery Anodes. The Journal of Physical Chemistry C,113(26), pp.11390–11398. doi.org/10.1021/jp901594g.