Nanomaterials are no longer just small—they are becoming smart. Across fields like medicine, electronics, energy, and materials science, researchers are now programming nanomaterials to behave in intentional, responsive ways.
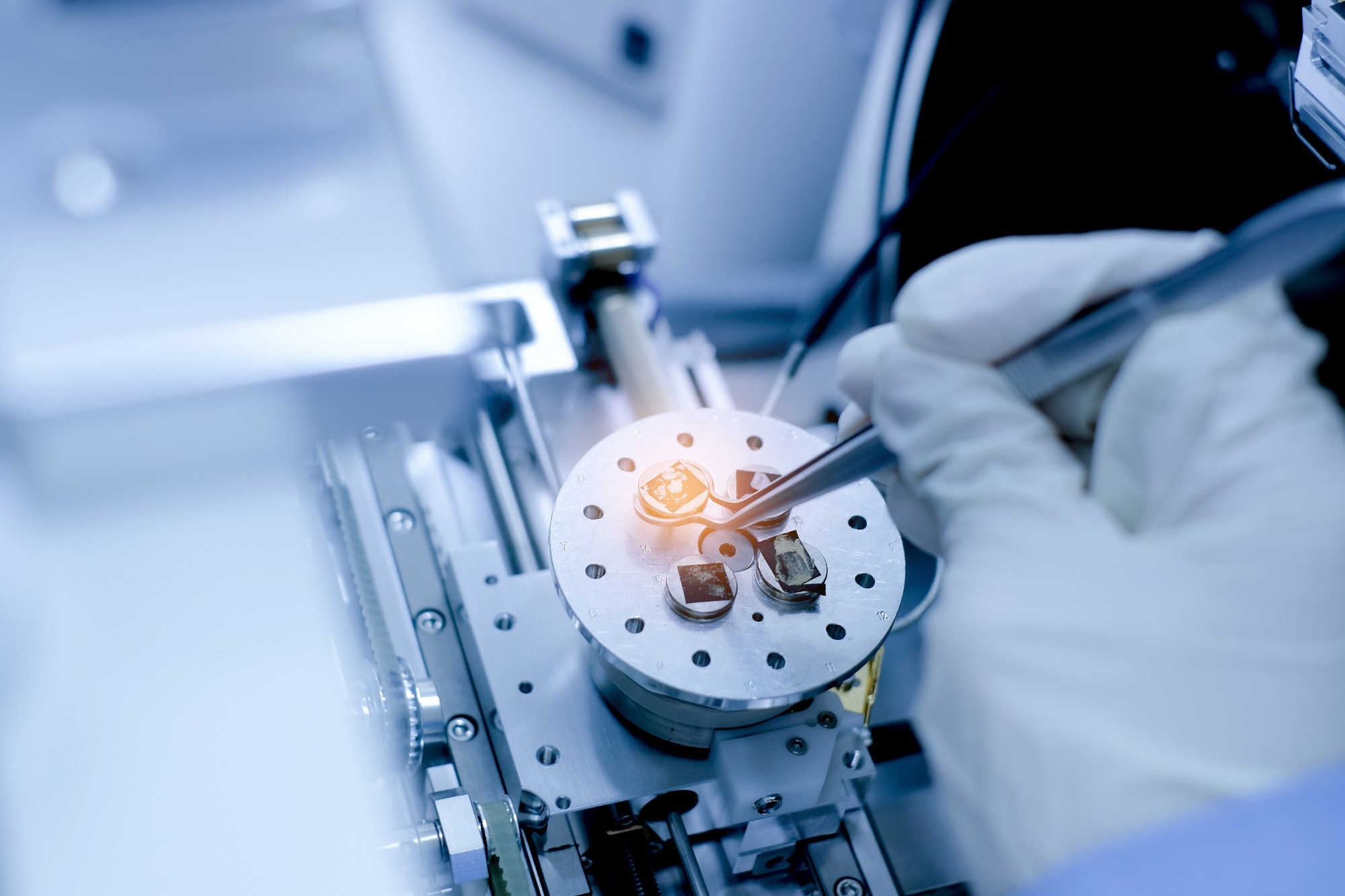
Image Credit: Anucha Cheechang/Shutterstock.com
These advanced materials are designed to detect specific stimuli, such as heat, pH changes, or light, and react with precise functions, like releasing a drug, changing structure, or switching conductivity. This capability unlocks major possibilities in areas ranging from targeted cancer therapies to adaptive electronics and wearable technologies.1
This progress raises key questions: How exactly do scientists program nanomaterials? What’s happening at the molecular level that allows these materials to act with purpose?
What Does "Programming" Nanomaterials Mean?
Programming nanomaterials means tuning their fundamental properties to control how they behave in different environments.2
This starts at the chemical level: scientists can design a material’s structure to define how it reacts, binds, or transforms under specific conditions. Surface functionalization adds further specificity by attaching molecules such as DNA strands, peptides, or polymers to a material’s surface, enabling selective interactions and triggered behaviors.3
Morphology—the size, shape, and surface texture of nanomaterials—is also crucial. Engineering particles into spheres, rods, cubes, or hollow structures can dramatically affect their optical, catalytic, and mechanical properties. Assembly strategies such as self-assembly and scaffold templating then organize these building blocks into ordered 1D, 2D, or 3D architecture, providing additional levels of structural complexity and functionality.2,3
A core feature of programmed nanomaterials is their ability to respond to external stimuli, including pH shifts, enzymatic activity, temperature changes, light, or chemical signals.3 This dynamic responsiveness underpins closely related fields.
- Stimuli-responsive materials physically or chemically change in response to external cues.
- Smart materials integrate sensing and actuation to autonomously adapt to changing conditions.
- Self-assembling nanostructures use molecular recognition or templating strategies to organize themselves into defined patterns.4
Emerging techniques like DNA-programmed assembly demonstrate how nanomaterials can be "instructed" to form highly ordered structures through bottom-up fabrication. By leveraging predictable DNA base-pairing, scientists can control spatial organization with nanometer-scale precision.4
Download your PDF copy now!
Mechanisms of Programming: How It’s Done
Programming nanomaterials involves a combination of molecular engineering, templating strategies, and the controlled use of external stimuli. Researchers use complementary approaches to design materials that change structure or function in response to specific conditions.
Each method supports distinct types of responsiveness, enabling tailored behavior for a range of applications.5
Surface Functionalization
Surface functionalization is a fundamental technique. By chemically attaching functional groups, polymers, or biological molecules to a nanoparticle’s surface, scientists can control how it interacts with other particles and its surroundings. Surface chemistry determines key attributes like binding selectivity, reactivity, and sensing ability.
For example, nanoparticles functionalized with DNA strands can self-assemble into highly programmable 2D and 3D architectures. These modifications enable the material to detect molecular cues, bind specific targets, or trigger structural changes.6
Encapsulation Within Nanocarriers
Encapsulation is another key programming technique. Here, active agents such as drugs, catalysts, or sensors are enclosed within nanoscale shells. These carriers are engineered to release their contents only when exposed to specific triggers like pH shifts, enzymatic activity, or temperature changes.
Encapsulation not only protects sensitive cargo but also provides a mechanism for smart delivery, where materials act only under particular biological or chemical conditions, reducing off-target effects.1,5
Science in 1 minute: What is microencapsulation for?
Responsive Polymers
Responsive polymers add another layer of programmability. These materials change shape, volume, or other physical properties in response to stimuli such as light, heat, electric fields, or mechanical stress.
They can be embedded into nanomaterials to create dynamic systems capable of reversible transformations. Shape-memory polymers and electroactive polymers, for instance, are used to build programmable surfaces and actuators that respond autonomously to environmental triggers.7
Self-Assembly
Self-assembly allows nanomaterials to spontaneously organize into ordered structures without external direction. This process relies on carefully designed interactions between components, often drawing on supramolecular chemistry or DNA-based recognition.6
It enables the creation of complex, hierarchically organized materials, including crystalline lattices, nanoparticle superstructures, and functionalized 3D networks. Innovations in DNA origami and templated polymer assemblies continue to expand what's possible with programmable nanostructures.6
External Triggers
External stimuli such as light, heat, magnetic fields, or electric fields are often used to program behaviour into nanomaterials post-assembly. Materials engineered with trigger-responsive elements can change color, conductivity, shape, or chemical activity on demand. For example, multi-beam optical interference can sculpt 3D nanomaterials with near-arbitrary complexity by controlling the spatial distribution of light.1, 5
Examples of Programmed Nanomaterials in Action
Targeted Drug Delivery
One of the most compelling applications of programmed nanomaterials is their use in targeted drug delivery systems—platforms designed to release therapeutic agents only under specific conditions, such as changes in pH or temperature. A notable example is the use of pH-responsive delivery systems, which exploit the acidic microenvironment typical of tumors to trigger drug release.8
Researchers have developed hydrogels and nanocomposites that remain stable at physiological pH but degrade or swell in mildly acidic conditions. This structural change enables the controlled release of their therapeutic cargo specifically at the tumor site.
For instance, Mazidi et al. demonstrated this approach using superparamagnetic iron oxide nanoparticles (SPIONs) embedded in a polyurethane nanofiber matrix and loaded with the chemotherapy drug doxorubicin (DOX). Their system showed a strong pH sensitivity, favouring drug release in the acidic environment of tumor tissues.8
Mathematical modeling of the system revealed a mix of non-Fickian and Fickian diffusion behavior, suggesting controlled, long-term drug delivery over more than 60 days. This environment-triggered release mechanism enhances treatment precision, improves therapeutic outcomes, and reduces the risk of off-target side effects.8
Self-Healing Materials
Programmed nanomaterials are also enabling a new generation of self-healing systems, with applications spanning both structural and electronic technologies.
For structural uses, microcapsule-based systems embedded in polymer composites have been widely developed. When damage occurs, the rupture of these microcapsules releases healing agents that autonomously repair cracks, restoring mechanical integrity and extending the material’s lifespan.9
In electronics, self-healing polymers have been created for devices such as organic field-effect transistors, energy storage systems, and flexible sensors. These systems often rely on dynamic chemical bonds, such as hydrogen bonding or π–π interactions, to recover both mechanical and electronic function after damage.9
For example, Munaoka et al. developed self-healing electrodes for lithium-ion batteries and showed that they improved cycling stability and safety by using nanomaterials capable of autonomously repairing microcracks.10
Light-Sensitive Nanoparticles
Another innovative use of programmed nanomaterials is in light-sensitive nanoparticles for photothermal therapy (PTT). These systems utilize upconversion nanoparticles (UCNPs) and X-ray nanoscintillators to convert deeply penetrating near-infrared (NIR) or X-ray light into heat or reactive oxygen species for localized cancer treatment.
UCNPs, such as NaYF₄ doped with Er³⁺ and Yb³⁺, absorb NIR light and emit visible or UV light, which activates photosensitizers attached to their surface or embedded within them. This activation generates localized heat or singlet oxygen, enabling noninvasive tumor ablation.11
Chen et al. reported successful in vivo tumour control using mesoporous silica-coated UCNPs loaded with photosensitizers and functionalized with folic acid for targeted delivery.12 Additional designs used orthogonal emission UCNPs, which could emit different wavelengths under separate NIR excitations, allowing programmable, stepwise treatments for improved therapeutic outcomes.11,12
Looking Ahead
While programmed nanomaterials hold enormous promise, challenges remain, such as scaling production, ensuring safety, and achieving consistent control in complex environments.
However, as fabrication techniques and molecular design tools advance, the range of applications continues to grow. From adaptive sensors that respond to real-time biological signals to precision therapies tailored to individual patients, these materials are laying the foundation for more responsive, intelligent systems.
With continued interdisciplinary research, programmed nanomaterials could redefine how we design, treat, and interact with the world around us.
To explore more innovations in smart and responsive materials, read:
References and Further Readings
1. Van Gough, D.; Juhl, A. T.; Braun, P. V., Programming Structure into 3d Nanomaterials. Materials today 2009, 12, 28-35. https://experts.illinois.edu/en/publications/programming-structure-into-3d-nanomaterials
2. Kahn, J. S.; Gang, O., Designer Nanomaterials through Programmable Assembly. Angewandte Chemie International Edition 2022, 61, e202105678. https://onlinelibrary.wiley.com/doi/abs/10.1002/anie.202105678
3. Yang, R. X.; McCandler, C. A.; Andriuc, O.; Siron, M.; Woods-Robinson, R.; Horton, M. K.; Persson, K. A., Big Data in a Nano World: A Review on Computational, Data-Driven Design of Nanomaterials Structures, Properties, and Synthesis. ACS nano 2022, 16, 19873-19891. https://pubs.acs.org/doi/10.1021/acsnano.2c08411
4. Luo, C.; He, L.; Chen, F.; Fu, T.; Zhang, P.; Xiao, Z.; Liu, Y.; Tan, W., Stimulus-Responsive Nanomaterials Containing Logic Gates for Biomedical Applications. Cell Reports Physical Science 2021, 2. https://www.sciencedirect.com/science/article/pii/S2666386421000357
5. Xie, M.; Gao, M.; Yun, Y.; Malmsten, M.; Rotello, V. M.; Zboril, R.; Akhavan, O.; Kraskouski, A.; Amalraj, J.; Cai, X., Antibacterial Nanomaterials: Mechanisms, Impacts on Antimicrobial Resistance and Design Principles. Angewandte Chemie International Edition 2023, 62, e202217345. https://pubmed.ncbi.nlm.nih.gov/36718001/
6. He, L.; Mu, J.; Gang, O.; Chen, X., Rationally Programming Nanomaterials with DNA for Biomedical Applications. Advanced Science 2021, 8, 2003775. https://advanced.onlinelibrary.wiley.com/doi/full/10.1002/advs.202003775
7. Waidi, Y. O., Recent Advances in 4d‐Printed Shape Memory Actuators. Macromolecular Rapid Communications 2025, 2401141. https://pubmed.ncbi.nlm.nih.gov/40014667/
8. Mazidi, Z.; Javanmardi, S.; Naghib, S. M.; Mohammadpour, Z., Smart Stimuli-Responsive Implantable Drug Delivery Systems for Programmed and on-Demand Cancer Treatment: An Overview on the Emerging Materials. Chemical Engineering Journal 2022, 433, 134569. https://ui.adsabs.harvard.edu/abs/2022ChEnJ.43334569M/abstract
9. Mashkoor, F.; Lee, S. J.; Yi, H.; Noh, S. M.; Jeong, C., Self-Healing Materials for Electronics Applications. International Journal of Molecular Sciences 2022, 23, 622. https://pmc.ncbi.nlm.nih.gov/articles/PMC8775691/
10. Munaoka, T.; Yan, X.; Lopez, J.; To, J. W.; Park, J.; Tok, J. B. H.; Cui, Y.; Bao, Z., Ionically Conductive Self‐Healing Binder for Low Cost Si Microparticles Anodes in Li‐Ion Batteries. Advanced Energy Materials 2018, 8, 1703138. https://www.scholars.northwestern.edu/en/publications/ionically-conductive-self-healing-binder-for-low-cost-si-micropar
11. Sun, B.; Teo, J. Y.; Wu, J.; Zhang, Y., Light Conversion Nanomaterials for Wireless Phototherapy. Accounts of Chemical Research 2023, 56, 1143-1155. https://pubmed.ncbi.nlm.nih.gov/36897248/
12. Chen, S.; Weitemier, A. Z.; Zeng, X.; He, L.; Wang, X.; Tao, Y.; Huang, A. J.; Hashimotodani, Y.; Kano, M.; Iwasaki, H., Near-Infrared Deep Brain Stimulation Via Upconversion Nanoparticle–Mediated Optogenetics. Science 2018, 359, 679-684. https://pubmed.ncbi.nlm.nih.gov/29439241/
Disclaimer: The views expressed here are those of the author expressed in their private capacity and do not necessarily represent the views of AZoM.com Limited T/A AZoNetwork the owner and operator of this website. This disclaimer forms part of the Terms and conditions of use of this website.