This article, related to the properties, synthesis, and applications of graphene, is meant to provide a broad understanding of these topics for both researchers and non-researchers alike.
.jpeg)
Graphene’s Atomic Structure
Graphene is rapidly finding its way into a wide range of applications and offers a number of benefits; for instance, it is used for developing innovative products as well as improving certain properties in currently available products. This article has been created to convey a general understanding of graphene, the different types of graphene available, and the methods of synthesis and applications of graphene.
What is Graphene?
An allotrope of carbon, graphene exists as a two-dimensional (2D) planar sheet and can be regarded as a single atomic graphite layer. Technically, graphene is a non-metal but it is usually known as a quasi-metal because its properties are similar to that of a semi-conducting metal. Therefore, graphene possesses a number of unique properties that cannot be found in other non-metallic materials. Each carbon atom is bonded covalently (sp2 hybridized) to three other carbon atoms in a hexagonal arrangement, leaving one free electron for each carbon atom. This single free electron exists in a p-orbital that sits above the material’s plane. Within the graphene sheet, each hexagon has two pi-electrons, which are delocalized and enable efficient conduction of electricity. In addition, the holes present in the structure enable phonons to pass through unhindered, which results in high thermal conductivity.
Since graphene has many unique properties, it is ideal for use in electronic applications when compared to other traditional materials. The most prevalent and significant property of graphene is its electrical conductivity.
Graphene lacks an electronic band-gap (which means it cannot be switched on or off) as the conduction and valence bands have a small overlap and the electrons serve as massless relativistic particles. At room temperature, the graphene material can show a concentration of charge carriers up to 1013 cm−2, with a mobility of 1 x 104 cm2 V−1 s−1. However, this can increase to 2 x 105 cm2 V−1 s−1 at low temperatures. Since the charge carriers function as quasi-particles, also referred to as massless Dirac Fermions, graphene also displays a half-integer Quantum Hall Effect (QHE). The QHE can be defined as the relationship of the density, charge, and velocity of the charge carriers. When a magnetic field is applied along the axis perpendicular to the plane of the conducting material, the QHE takes place. Under these situations, the path of the carriers becomes curved, causing an accumulation of opposite charges at both ends of the material.
Since graphene is a two-dimensional material, the electron confinement creates distinct band levels called Landau levels, which are filled by the charge carriers. These levels are only half-filled by the charge carriers in graphene, unlike other materials. This leads to a quantization of the Landau levels, and in effect the graphene’s energy levels.
Graphene is also known to possess good mechanical, thermal, and optical properties. Although single-sheet graphene is a highly transparent material, each layer in thickness can absorb up to 2.3% of white light, with a reflectance of less than 0.1%. Additionally, there is an increase in linear absorbance with regards to the number of layers stacked on top of each other. At room temperature, a suspended graphene sheet can show a thermal conductivity of 3000–5000 W m−1 K−1, but when it is fixed to another substrate, the thermal conductivity can drop down to 600 W m−1 K−1. The drop is induced by a scattering of phonons at the interface which obstructs their movement, while the phonon path is not interrupted in free-standing graphene. Even at this lower conductivity, the thermal conductivity continues to be twice as large as copper. Furthermore, graphene is one of the strongest materials ever made, with a single-layer graphene withstanding up to 42 N m−1 of stress, with a Young’s modulus of 1.0 TPa.
Types of Graphene
.jpeg)
A Graphene Sheet
Real graphene has a thickness of a single atomic layer (often known as a monolayer). While this material usually exists as a film, it can be floated off the substrate and can be deposited again onto another substrate or employed in its isolated form. However, there are different types of graphene that contain powder-form materials, such as graphene nanoribbons, graphene nanoplatelets, graphene oxide, graphene quantum dots, and even graphene-enabled products like graphene masterbatches or graphene ink.
Graphene can be synthesized by three different ways. They are as follows:
While graphene can be completely synthetic, those techniques have not been demonstrated to be commercially viable.
Chemical Vapor Deposition (CVD) Graphene Films
.jpg)
A Monolayer Graphene Film
Different methods can be used to produce graphene films. These include chemical reduction, epitaxial growth, and mechanical and thermal exfoliation, but among these, chemical vapor deposition (CVD) is the most common method that is currently used in production. In the CVD method, volatile gas molecules are combined and deposited onto a substrate. The process occurs in a reaction chamber, where the material is formed on the substrate’s surface and the waste gases are pumped out. Here, temperature dependence plays an important role and can influence the type of reaction that takes place. While ultra-pure, high-quality graphene films are produced by the CVD process, the by-products that are created during the reaction can be harmful because of the volatile nature of the precursor gases.
CVD creates the graphene film in two steps. In the first step, a precursor material is pyrolyzed to create carbon atoms on a substrate material. When the material is pyrolyzed on the substrate, it prevents the formation of carbon clusters. High heat is required because of the amount of energy needed to break the carbon bonds (C-C = 347 kJ mol−1, C=C = 614 kJ mol−1, C≡C = 839 kJ mol−1, C-H = 413 kJ mol−1), and as a result, a metal catalyst is needed during the process. The next step is a heat-intensive step in which the dissociated carbon atoms are assembled onto a substrate (in the presence of a catalyst), which creates a single-layer structure.
It has been predicted that CVD graphene films have strong mechanical, chemical, magnetic, and electronic properties based on the arrangement of the atoms in the film. However, only a few experiments have been produced to analyze these interactions because of the five-order-of-magnitude difference between the atoms and grains at grain boundaries.
Copper is one substrate that is known to create high-quality graphene. It functions both as a substrate and as a catalyst. When copper bonds to the carbon atoms, it provides strong interactions between the carbon and substrate, enabling a single graphene layer to be easily formed on the surface. Between the layers of graphene, copper oxide can also be inserted, which makes it easy to remove a single layer. When the copper substrate is treated, the surface morphology of the substrate-catalyst can also be rearranged. This is known to produce graphene with fewer defects.
Graphene Oxide (GO)
.jpg)
Graphene Oxide
The oxidation of graphite oxide produces graphene oxide (GO). The oxidation process is advantageous because it functionalizes the surface of the graphene layers with many species of oxygenated functional groups. An enhanced layer separation and improved hydrophilicity are provided by the multiple functional groups. The hydrophilicity enables the graphene oxide to experience ultrasonic irradiation, which creates a single or a few graphene layers, which are highly stable when dispersed in DI Water and other solvents.
GO is known to have many desirable properties. For example, it disperses very easily in numerous mediums such as organic solvents, aqueous solvents, and various matrices. The presence of an electron-rich graphene backbone as well as electron-rich oxygen species enables further surface functionalization, which results in an adaptable material for multiple applications. However, GO is an electrical insulator and is prone to low electrical conductivity. GO is also soluble in many solvents, both organic and aqueous.
.gif)
Graphene Oxide is Highly Soluble
In order to realize the advantages, GO is usually dispersed, added into a formulation, fabricated into a film or other nano-enabled product, and then finally reduced to restore the structure of graphene.
Reduced Graphene Oxide (rGO)
Many approaches are available to reduce GO into reduced graphene oxide (rGO), but a majority fall into three key categories: thermal reduction, chemical reduction, and electrochemical reduction. The other techniques include annealing, hydrazine vapor treatment, laser and microwave reduction. The reduction process is important to creating rGO, as it establishes how consistent the rGO structure is with the GO precursor. Numerous commercial producers of graphene nanoplatelets are actually providing a product similar to industrial scale rGO as their GNP product. However, this technique varies from the rGO most people refer to which is a superior quality research product that is used for nano-enabled devices.
Chemical reduction is a scalable technique but can often only provide poor yields and utilizes very toxic materials such as hydrazine. rGO produced by this technique usually displays a low surface area and has a low conductivity than the GO precursor.
Thermal reduction creates rGO with a high surface area that is close to the surface area of pristine graphene. However, the strong heating process causes a high-pressure build-up of carbon dioxide which induces structural damage to the graphene layers. The structural defectiveness can then lead to a reduction in the total mass (and yield against the theoretical output), voids, vacancies, and it can obstruct the mechanical strength of the material.
Electrochemical reduction delivers the best results with regards to production and quality. The rGO produced is consistent with that of pristine graphene. In the electrochemical process, the substrates (usually glass or ITO) are coated with a layer of GO and a current is conveyed through the material (via electrodes at either end of the substrate). rGO produced by this technique have exhibited a high carbon to oxygen ratio and have shown conductivity comparable to that of silver. The process also benefits from zero toxic waste. This process does, however, suffer from issues relating to the feasibility of the scalability of the technique.
Graphene Nanoplatelets
.jpg)
Graphene Nanoplatelets
Graphene nanoplatelets (GNPs) are usually synthesized by micromechanical cleavage of bulk graphite and can only yield graphene flakes in limited quantities which are blended with graphitic stacks. Mass GNP production regularly uses mechanical cleavage followed by chemical reduction to make the final GNP product.
Another technique to mass produce GNPs is by plasma exfoliation. A unique advantage of plasma exfoliation is the ability to synthesize and functionalize the GNPs to boost dispersion in the host matrix in one dry processing step. The RF or Microwave Plasma Reactor has a vacuum applied to eliminate atmospheric contaminants as well as residual contaminants which are expelled during the plasma milling process. These advantages make the material a superior choice for mass industrial applications with a wide range of available functional groups such as OH, COOH, N2, NH2, and F.
Owing to the lower cost of capital equipment, input materials, and plasma purification and/or functionalization, the GNPs can eventually be a cheaper material than CNTs on a ton level thereby enabling increased industrial applications with early adopters.
Graphene and Graphene Oxide Quantum Dots
.png)
Graphene Quantum Dots
Graphene and graphene oxide quantum dots (GQDs) can be synthesized into a variety of forms, from single-layer to tens of layers, but are usually less than 30 nm. GQDs also display similar properties to other types of quantum dots. Similar to many graphene-based materials, GQDs display a good linear dispersibility, a large surface area, and a high charge carrier mobility. GQDs also display an excellent hole transporting ability, making them efficient materials for hole-transport layers. They are beneficial materials for both opto-electronic and electronic applications.
GQDs can currently be made by numerous techniques which include both top-down and bottom-up methods. Production by bottom-up techniques can create GQDs with a controlled size (because of the ability to regulate the band gap), but the synthesis itself can be complex which requires rigid conditions. Top-down approaches are in their early stages, but do provide a much cheaper and simpler approach. The breakdown of graphite oxide can create single layered GQDs, but the yields are not high and the size distribution cannot be easily regulated. GQDs can also be synthesized by the breakdown of a range of carbon allotropes including fullerenes, CNTs, and carbon fibers. Other techniques include ultrasonication, radical methods, microwave irradiation, and hydro-thermal or solvo-thermal methods. The key challenge that faces GQD production presently is finding an efficient process that can advance the production to industrial levels, as they have the potential for many applications.
The applications of GQDs include supercapacitors, lithium-ion batteries, and solar cells. But one area in which they are mainly beneficial is in LED displays. GQD-LEDs are a growing area and have been created by etching CVD-grown graphene with block copolymers, which is followed by fabrication with graphite intercalating compounds. The process makes graphene flakes that are low in oxygenated functional groups, of low toxicity and environmentally safe. It is also both an inexpensive and scalable process. GQDs discharge light upon excitation, and so are currently being tested as emitters in organic light-emitting diodes (OLEDS). Thus far, they have displayed a luminescence of 1000 cdm−2. The thin nature of GQDs may also pave the way for future opportunities to create and perfect foldable displays.
Graphene Nanoribbons
.jpeg)
In contrast to many other forms of graphene, which are 2D, graphene nanoribbons (GNRs) are quasi-one-dimensional materials possessing an ultra-thin width. The electrical properties that GNRs display are very tunable and can be tuned by edge morphology, dimension confinement, and functionalization of the GNR. GNRs also display large aspect ratios, a conductive matrix, a high surface area, and good mechanical flexibility. The combination of these properties has bestowed GNRs a robust footing in composite materials for electronic applications. GNR anode composites have been revealed to exhibit a specific reversible capacity of 1130 mAh g−1, with a coulombic efficiency >98%.
GNRs are created by many approaches. One of the most common approaches involves unzipping the walls of MWCNTs with sodium and potassium-based compounds, sonicating and drying under vacuum. They can also be formed by plasma etching of nanotubes onto polymer films, epitaxial growth and annealing on silicon carbide and CVD.
.gif)
Graphene Nanoribbons Band Gaps are Controllable
GNRs can improve the performance of lithium-ion batteries via edge chirality effects. GNRs consist of a band gap that is inversely proportional to their width, which is reliant on their edge chirality. Chirality occurs at the edges as the electron confinement potential deforms the wave-function and causes the electrons to move in a single direction, with more weight in the ‘positive x’ direction. This results in a current in the ‘positive y’ direction. For electronic applications, the edges in GNRs have exhibited the best results when armchair and metallic edges are present because of their semi-conducting abilities. Armchair edges also decrease the band gap energy when there is a defined width. The energy at the edges of GNRs is proportional to their density and armchair edges are more firmly packed at the graphene interface, so the energy is higher than that of zig-zag edges. Such edges can be formed on GNRs by electron beam lithography and electron beam irradiation. A GNR with a high concentration and purity of armchair edges has been found to provide very efficient p-n junctions in electronic gadgets.
Graphene Aerogels
.jpeg)
A Graphene Aerogel Foam Sits on a Flower
Carbon aerogels are derived by sol-gel synthesis techniques and are a unique group of high-surface-area materials. Their high mass-specific surface area, environmental compatibility, electrical conductivity, and chemical inertness make them highly promising materials for numerous energy-related applications. Recent developments in manipulating their morphology make them particularly well-matched for super-capacitor applications.
Aerogels are a special class of open-cell foams that display many unique and remarkable properties, such as continuous porosity, low mass density, and high surface areas. These properties are resulting from the aerogel microstructure, which consists of 3D networks of interconnected nanometer-sized particles. Aerogels are usually prepared using sol–gel approaches, a process that converts molecular precursors into highly cross-linked inorganic or organic gels that can then be dried using methods such as freeze drying, supercritical drying, etc. to preserve the weak solid network. In the case of organic and carbon aerogels, the transformation includes the polymerization of multi-functional organic species into 3D polymer networks.
Graphene Masterbatches
.jpeg)
Graphene masterbatches are composite materials that have a graphene-based compound (most frequently GO) and a polymer. The graphene is used to improve the properties of a range of common polymeric materials. Many polymers display desirable properties such as low toxicity, bio-compatibility, low cost, and chemical resistance, but they lack the necessary mechanical properties. By adding graphene into polymer matrices, the polymers keep their original properties but benefit from improved stiffness and rigidity, while still being lightweight.
Using graphene as a filler compound instead of conventional inorganic materials can bring an improved electrical conductivity to the polymer, but it does have some problems. In a number of graphene-based composites, graphene oxide serves as the dispersing support for other ions and molecules. In polymer masterbatches, this can result in problems as graphene doesn’t always disperse well in polymer phases (particularly polyolefins) because of a lack of positive interactions at the graphene-polymer interface. However, this can be handled by the use of a surfactant, or by modifying surface functionality of the graphene surface. The surfactant increases the surface interaction between the graphene and polymer. If functionalized, the functional groups boost interaction between itself and the polymer molecules. If the functional groups are not compatible, one may witness “islands of masterbatch” with easily observed islands of polymer in between well-dispersed graphene-polymer masterbatches.
Properties of Graphene
Graphene has many unique and necessary properties because of its all carbon structure and nanoscale geometry.
Electronic Properties
.jpeg)
Flexible Graphene Transistors
As graphene has a delocalized pi-electron system across the totality of its surface, the movement of electrons is extremely fluid. The graphene system also displays no band gap, because of overlapped pi-electrons, allowing for a smooth movement of electrons without the need to input energy into the system. The electronic mobility of graphene is very high and the electrons act like photons, in relation to their movement capabilities. The electrons are also able to travel sub-micrometer distances without scattering. From tests conducted thus far, the electron mobility was found to be in excess of 15,000 cm2 V−1 s−1, with the potential of producing up to 200,000 cm2 V−1 s−1.
Thermal Properties
.jpeg)
The repeating structure of graphene makes it a suitable material to conduct heat in plane. Interplane conductivity is challenging and normally other nanomaterials such as CNTs are added to increase interplane conductivity. The regular structure allows phonons to travel through the material without obstacle at any point along the surface. Graphene can display two types of thermal conductivity- inter-plane and in-plane. The in-plane conductivity of a single-layered sheet is 3000-5000 W m−1 K−1, but the cross-plane conductivity can be as low as 6 W m−1 K−1 because of the weak inter-plane van der Waals forces. The particular heat capacity for graphene has never been directly measured, but the specific heat of the electronic gas in graphene has been estimated to be about 2.6 μJ g−1 K−1 at 5 K.
Mechanical Strength
.jpeg)
Graphene’s Strength Enables High Performance Composites
Graphene is one of the sturdiest materials ever discovered possessing a tensile strength of 1.3 x 1011 Pa. Besides having an unsurpassed strength, it is also extremely lightweight (0.77 mg m−2). The graphene’s mechanical strength is unrivaled and as such can considerably improve strength in a number of composite materials.
Flexibility/Elasticity
The repeating sp2 hybridized backbone of graphene molecules permit flexibility, as there is rotation around few of the bonds, whilst still providing sufficient rigidity and stability that the molecule can endure variations in conformation and support other ions. This is a highly desirable property as there are not many molecules that can be flexible and supportive at the same time. With regards to its elasticity, graphene has found to possess a spring constant between 1 and 5 N m−1, with a Young’s modulus of 0.5 TPa.
Applications of Graphene
Graphene is a ground-breaking material. It has numerous applications substituting conventional materials as well as the ability to support applications formerly not possible before the arrival of 2D materials. The applications of graphene are truly infinite and many are yet to be discovered.
Sensors
.jpeg)
Types of Graphene Sensors
The perfect sensor can detect miniscule changes in its surrounding environment. Due to the planar and consistent arrangement of atoms in a graphene sheet, all the atoms within the sheet are exposed to the surrounding environment. This allows graphene to efficiently detect variations in its surroundings at micrometer dimensions, offering a high degree of sensitivity. Graphene is also able to detect separate events on a molecular level. A number of graphene’s properties are advantageous in sensor applications; as such, graphene could be used in sensors in a range of fields including diagnostics, DNA sensors, field effect transistors, bio-sensors, and gas sensors, to list a few.
Batteries
.jpeg)
Graphene-Lithium-Sulfur Battery
Graphene can be added into both the anode as well as the cathode in a range of battery systems to boost the efficiency of the battery and enhance the charge/discharge cycle rate. The exceptional electrical conductivity, surface area, and dispersibility of graphene improve the useful properties found in many traditional inorganic-based electrodes, whilst simultaneously relieving the electrodes of their limitations. As a result of its versatile nature, graphene has been added to lithium-ion batteries, supercapacitors, lithium-sulfur batteries, and fuel cells, of which there are many variations of each available on the market today.
Electron Emission Displays
.jpeg)
Graphene Displays
Graphene is an ideal material for use in electron emission displays as it displays a high aspect ratio and the dangling bonds at either end of the sheet permit for efficient electron tunneling. The linear disparity that the graphene surface provides creates massless Dirac Fermions. When exposed to an electric field, the field emission liberated electrons avoid all back-scattering as their escape velocity is autonomous to their energy. Graphene can turn-on an electric field at 0.1 V µm−2, with a field enhancement factor of up to 3700. This can go up to 4500 in screen printed graphene films.
Structural Composites
Graphene is added into a range of composites for applications where weight and strength are limiting factors, for instance in the aerospace sector. Graphene is being added to numerous materials to make the current material more lightweight and stronger. For the aviation sector, a composite material which is much lighter than steel but will still provide the required strength will save plenty of money on fuel consumption, which is why graphene is now being combined into such materials. Graphene-based structural composites have a massive potential to become an extensively used substitute for many materials used at present.
Catalyst Supports
.jpeg)
Graphene Catalyst Supports
Despite the fact the surface of graphene is planar and uniform, like any other material in existence it is subject to inherent defects. Catalysts in the form of metal ions can rest in these cavities and be supported. Besides providing mechanical support, the outstanding charge carrier ability of graphene assists the charge transfer reactions including the catalyst. Graphene is also inert and does not inhibit (in a negative way) the interaction between the catalyst and the substrate materials. Graphene also provides a uniform dispersion of catalyst particles, so the catalyst-substate reaction is dependable across the entire support.
Polymer Masterbatches
Graphene can be added into polymeric materials to produce graphene-polymer composite materials. As a number of polymeric materials suffer from strength-related issues, the combining of graphene can help to boost the tensile strength of the polymers, prolonging the shelf life of the polymeric material in commercial applications. Adding graphene into polymers can also offer polymers electrical conductivity properties.
Functional Inks
Graphene can be used in functional inks for electronic, anti-corrosion, and heat resistant purposes. By combining graphene into ink formulations, the conductivity properties related to graphene influence the ink, causing it to turn conductive. The inks can subsequently be used to coat electronics. Graphene is non-toxic, environmentally friendly, cheaper, quick-drying and recyclable than other conducting inks. Graphene also possesses a high thermal stability, making it suitable for heat-resistant ink coating in electronic applications that create large amounts of heat. It is also an ink of choice in cases where processing temperatures have to be high, as the graphene won’t break down during the manufacturing process. Graphene also displays superior chemical stability and is inert. For applications where environmental factors are a problem, graphene inks can provide a consistent barrier to safeguard materials from chemicals and corrosion.
Sources and Further Reading
- Huang X., Xiaoying Q., Boey F. and Zhang H., Graphene based composites, Chem Soc. Rev., 2012, 41, 666-686
- Zhou G., Yin L., Wang D. and Cheng H., A fibrous hybrid of graphene and sulfur nanocrystals for high performance lithium-sulfur batteries, ACS Nano, 2013, 7(6)
- Cheng Q., Tang J., Zhang H., Graphene and carbon nanotube composite electrodes for supercapacitors with ultra-high energy density, Phys. Chem. Chem. Phys., 2011, 13, 17615-17624
- Peng Z., Xiang C., Yan Z., Natelson D., Graphene Nanoribbon and Nanostructured SnO2 Composite Anodes for Lithium Ion Batteries, ACS Nano, 2013, 7(7)
- Haegyeom K., Dong-Hwa S., Sung Wook None K., Kisuk K., Highly reversible Co3O4/graphene hybrid anode for lithium rechargeable batteries, Carbon, 2011, 49(1), 326-332
- Bak S., Kim D., Lee H., Graphene quantum dots and their possible energy applications: A review, Current Applied Phyics, 2016, 11, 1192-1201
- Liu Y., Dobrinksy A., Yakobson B. I., Graphene edge from armchair to zigzag: The origins of nanotube chirality, Phys. Rev. Lett., 2010, 105, 235502
- Begliarbekov M., Sasaki K., Sul O., Yang E., Strauf S., Nano Lett., 2011, 11(11), 4874-4878
- Pop E., Varshney V., Roy A., Thermal properties of graphene: Fundamentals and applications, MRS bulletin, 2012, 37, 1273-1281
- Lei W., Li C., Cole M., Qu K., Ding S., Zhang Y., Warner J., Zhang X., Wang B., Milne W., A graphene -based large area surface-conduction electron emission display, Carbon, 2013, 56, 255-263
- www.cheaptubes.com
- www.graphenea.com
- http://www.businesswire.com/news/home/20161101006012/en/Global-Graphene-Battery-Market-Worth-USD-115
- https://kis.kaist.ac.kr/?mid=KINC_Highlight&highlight_item_srl=40620&catelevel_1_sel=733&catelevel_2_sel=737
- http://mceuengroup.lassp.cornell.edu/sites/mceuen/files/publications/JVSTB_Pushing_Graphene.pdf
- https://arxiv.org/ftp/arxiv/papers/1301/1301.6181.pdf
- http://www.graphene-info.com
- http://www.cam.ac.uk/research/news/new-graphene-based-inks-for-high-speed-manufacturing-of-printed-electronics
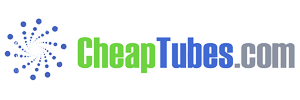
This information has been sourced, reviewed and adapted from materials provided by Cheap Tubes Inc.
For more information on this source, please visit Cheap Tubes Inc.