Viral outbreaks impact global health and healthcare systems; researchers and healthcare professionals advocate using antiviral agents to decrease the spread of viral pathogens. Research published in the journal Emergent Materials has explored the antiviral properties of intermetallic nanoparticles incorporated into polymeric fibers.
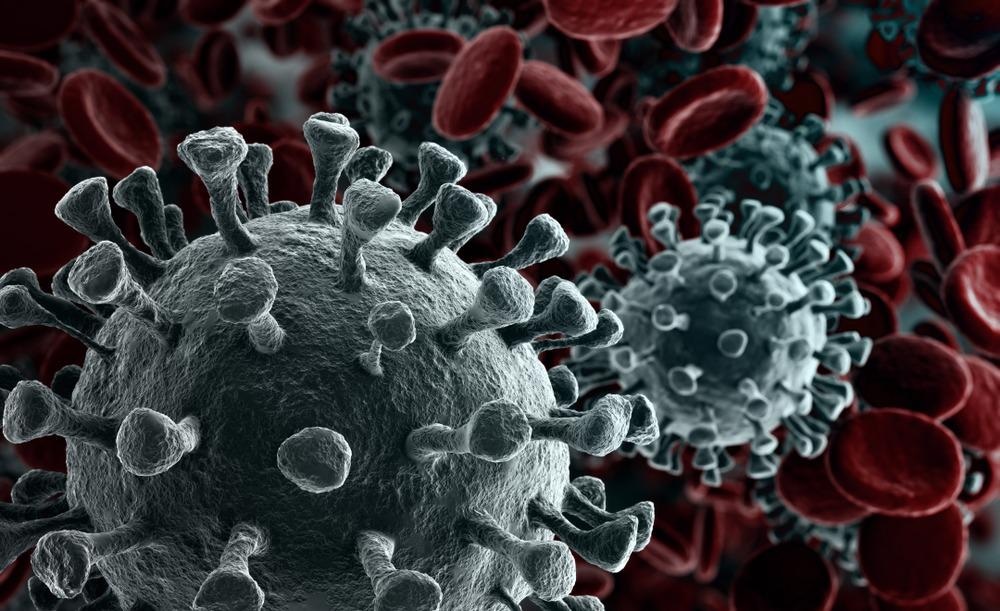
Image Credit: creativeneko/Shutterstock.com
Viruses are the most abundant life form, spreading easily between individuals through direct or indirect contact to cause infectious disease. Their core genetic material is composed of RNA or DNA, surrounded by a proactive protein coat.
There are numerous vaccines designed against viruses, with some leading to the eradication of viral infections like measles and smallpox. However, there are still many pathogens with no available treatment.
Scientists and pharmaceuticals turn to antiviral agents, which reduce the spread and likelihood of the viruses’ causing infections.
Nanomaterials have the potential to be used in infection treatment as they possess antimicrobial properties.
Intermetallic materials, a kind of metallic alloy composed of two or more elemental metals, exhibit strong antimicrobial properties, particularly copper-based alloys.
This research analyzed the antiviral properties of copper-zinc and copper-silver alloy composites for the first time, as both zinc and silver demonstrated promising antimicrobial activity. Previous work has often concentrated on analyzing the antiviral properties of single elemental nanoparticles.
Experiments were performed in bacteriophages, viruses that infect bacterial cells, as they are showcase structural features similar to animal and human viruses.
Methodology
The researchers obtained intermetallic copper-silver and copper-zinc nanoparticles. Morphologies were examined with scanning electron microscopy (SEM), and images were processed with built-in software.
Fourier-transform infrared (FTIR) spectra of the nanoparticles were obtained with a PerkinElmer Frontier FT-IR/FIR spectrometer, and Raman spectra were acquired by a Renishaw inVia Raman microscope. The samples were prepared for elemental and metal trace analysis.
The study used Escherichia coli bacteriophage T4 (to model DNA viruses) and Escherichia coli bacteriophage MS2 (to model RNA viruses) as the model organisms. The viruses were cultured and antiviral activity was determined.
Nanocomposite fibers were developed from poly(methyl methacrylate) (PMMA) employing pressurized gyration. Copper-silver and copper-zinc nanoparticles were introduced into the fibers, and antiviral properties of the fibers were analyzed against bacteriophage T4.
Results
SEM analysis revealed the morphology of the two intermetallic nanoparticles.
Intermetallic copper-silver and copper-zinc nanoparticles are shown in Figure 1 to be spherical shaped and are agglomerated. The average size of copper-silver was found to be 90–95 nm and copper-zinc was 100–120 nm.
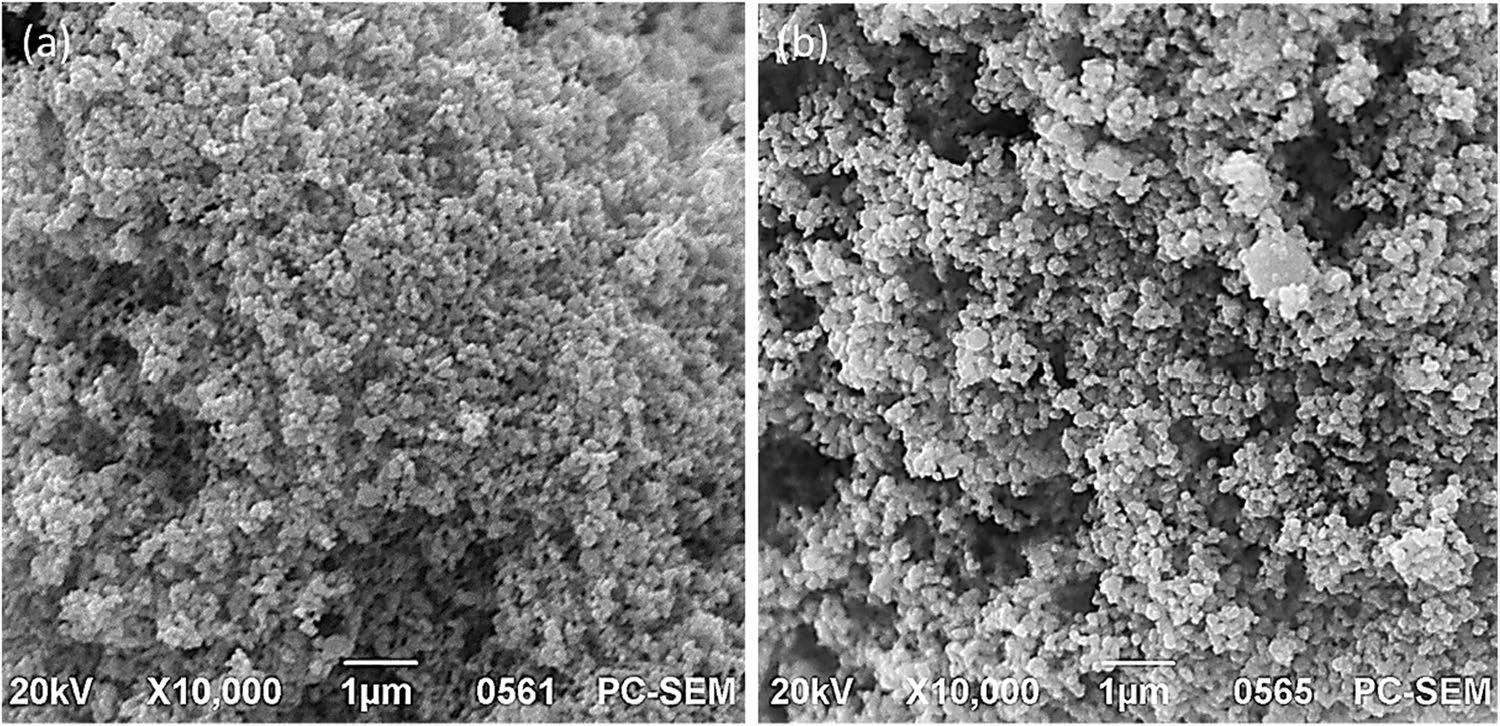
Figure 1. Scanning electron micrographs of (a) copper-silver and (b) copper-zinc nanoparticles. Image Credit: Matharu, et al., 2021. Image Credit: Matharu, R. K., et al (2021)
Figure 2 displays both FTIR (red) and Raman (blue) spectra of the intermetallic nanoparticles.
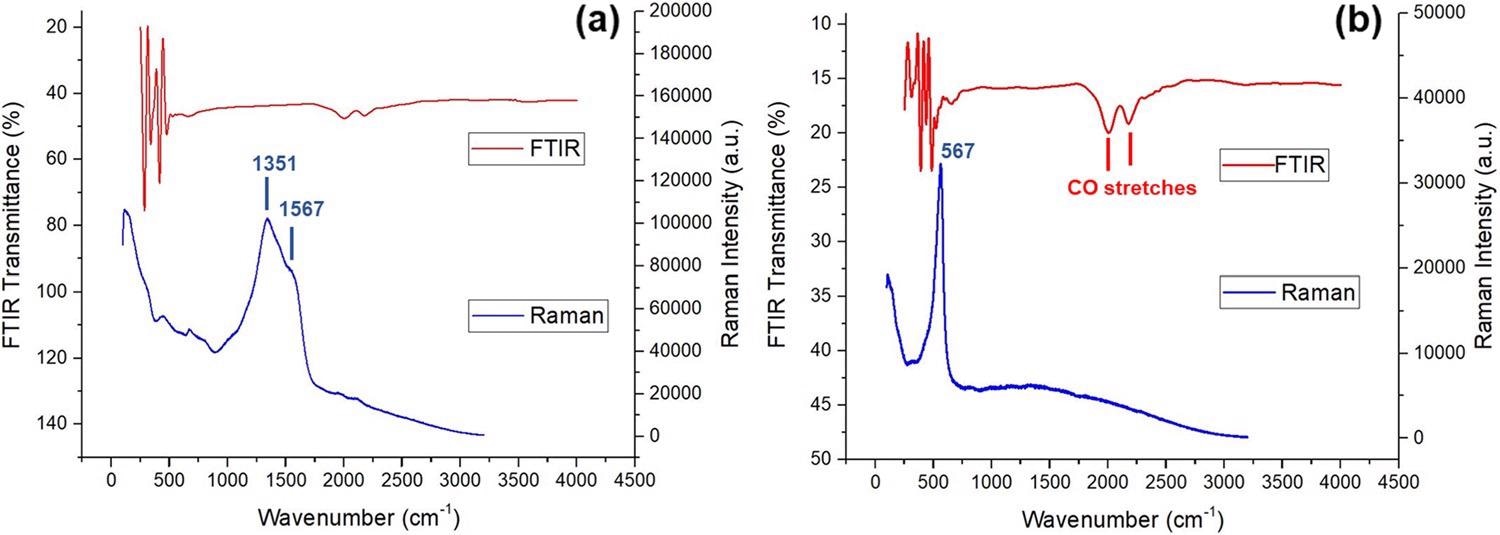
Figure 2. FTIR and Raman spectra of (a) copper-silver and (b) copper-zinc nanoparticles. Image Credit: Matharu, et al., 2021
Detectable amounts of metal ions and concentrations of major ions (silver, copper, and zinc) evaluated from wholly digested copper silver and copper-zinc samples are displayed in Table 1.
Table 1. ICP-OES metal trace and elemental analysis of intermetallic copper-silver (CuAg) and copper-zinc (CuZn). Source: Matharu, et al., 2021
ICP digested sample |
CuAg |
CuZn |
Traced ion |
Ca, Mn, Zn, Br |
Fe, Ni, W, Br |
Main ion |
Cu |
Ag |
Cu |
Zn |
Mean (ppm) |
0.08035 |
5.752 |
4.1860 |
2.2561 |
wt% |
1.38 |
98.62 |
65.0 |
35.0 |
Mole ratio |
0.022 |
0.914 |
1.023 |
0.535 |
Calculated formula |
Cu0.60Ag25 |
Cu21Zn11 |
Standardised formula |
CuAg42 |
Cu2Zn |
The viricidal properties of copper-zinc and copper-silver nanoparticles were evaluated against bacteriophages T4 and MS2. The number of infectious viral particles was quantified by a plaque assay before and after treatment.
Figure 3 depicts the antiviral activity of all concentrations analyzed. Researchers found that the nanoparticles were highly effective against bacteriophage MS2 rather than bacteriophage T4.
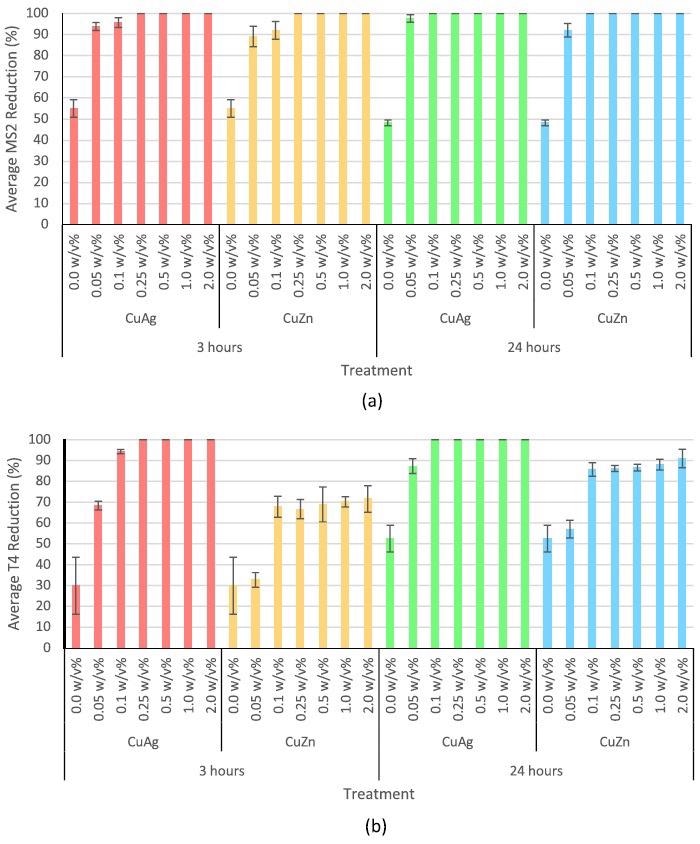
Figure 3. Antiviral activity of copper-silver and copper-zinc intermetallic nanoparticles at 0.05, 0.1, 0.25, 0.5, 1.0, and 2.0 w/v% against (a) bacteriophage MS2 for 3 and 24 hours and (b) bacteriophage T4 for 3 and 24 hours. Error bars represent standard deviation. 0.0 w/v% is the negative control. Image Credit: Matharu, et al., 2021
Copper-silver intermetallic nanoparticles, in particular, were highly potent against bacteriophage T4 than copper-zinc nanoparticles.
Figure 4 displays the antiviral properties of nanocomposite polymeric fibers when evaluated against T4 bacteriophage.
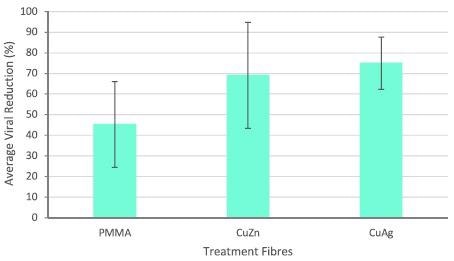
Figure 4. Antiviral activity of intermetallic nanocomposite fibers after 3 hours incubation. Error bars represent standard deviation. Negative control represents pure PMMA fibers. Image Credit: Matharu, et al., 2021
Discussion
Nanoparticles used in these investigations were characterized by various techniques. For instance, SEM analysis exposed that copper-zinc intermetallic nanoparticles were somewhat bigger than copper-silver nanoparticles, while FTIR analysis revealed that copper-zinc and copper-silver were free from organic contaminants.
Antiviral activity tests revealed that the nanoparticles were more efficient against RNA viruses when compared to DNA viruses; however, this analysis needs further evaluation.
The presence of zinc or silver in the compounds is attributed to a difference in potency. The viricidal activity of the intermetallic nanoparticles depends on the nanoparticles’ interaction with viral envelope glycoproteins, which inhibits viral diffusion and penetration into the host cell.
Intermetallic copper-silver nanoparticles exhibit greater antiviral properties when compared to single elemental nanoparticles, supported by previous and current research. Copper-zinc intermetallic nanoparticles showed synergistic antiviral properties of all three concentrations analyzed.
Antiviral agents and carrier polymeric matrices enhance the usability of the material enabling its use in commercial, industrial, and consumer applications. For this reason, copper-zinc and copper-silver intermetallic nanoparticles were introduced into polymeric fibers to evaluate their efficacy against viruses.
It was noticed that pure polymer (PMMA) fibers exhibited a moderate decrease in virions. Fibers with copper-silver nanoparticles showed slightly greater antiviral properties compared to fibers having copper-zinc intermetallic nanoparticles. The toxicity of intermetallic nanoparticles against viruses is evident, but its effect on human cells needs further investigation.
Conclusion
The current study details the antiviral properties of intermetallic copper-zinc and copper-silver nanoparticles. Antiviral analyses showed that both nanoparticles are effective against RNA viruses, while copper-silver nanoparticles were much potent against DNA viruses.
Also, their ability to be effective when introduced into polymeric fibers shows the potential of these nanoparticles in numerous applications, from environmental to biomedical engineering.
Continue reading: NANO-LLPO: Using Nanomaterials to Heal Wounds.
Journal Reference:
Matharu, R. K., Cheong, Y.-K., Ren, G., Edirisinghe, M., Ciric, L. (2021) Exploiting the antiviral potential of intermetallic nanoparticles. Emergent Materials. Available at: https://doi.org/10.1007/s42247-021-00306-2
References and Further Reading
- Bamford, D. H., et al. (2005) What does structure tell us about virus evolution? Current Opinion in Structural Biology, 15(6), pp. 655–663. doi.org/10.1016/j.sbi.2005.10.012.
- Bergh, Ø., et al. (1989) High abundance of viruses found in aquatic environments. Nature, 340(6233), pp. 467–468. doi.org/10.1038/340467a0.
- Wommack, K E & Colwell, R R (2000) Virioplankton: viruses in aquatic ecosystems. Microbiology and Molecular Biology Reviews, 64(1), pp. 69–114. doi.org/10.1128/MMBR.64.1.69-114.2000.
- Fongaro, G., et al. (2013) Evaluation and molecular characterization of human adenovirus in drinking water supplies: viral integrity and viability assays. Virology Journal, 10(1), pp. 166. doi.org/10.1186/1743-422X-10-166.
- Kukkula, M., et al. (1997) Waterborne outbreak of viral gastroenteritis. Scandinavian Journal of Infectious Diseases, 29(4), pp. 415–418. doi.org/10.3109/00365549709011840.
- Lee, S-H & Kim, S-J (2002) Detection of infectious enteroviruses and adenoviruses in tap water in urban areas in Korea. Water Research, 36(1), pp. 248–256. doi.org/10.1016/S0043-1354(01)00199-3.
- Reynolds, K A (2004) Adenovirus: balancing water treatment challenges. Water conditioning and purification. Available at: https://wcponline.com/2004/09/15/adenovirus-balancing-water-treatment-challenges/.
- Van Heerden, J., et al. (2003) Incidence of adenoviruses in raw and treated water. Water Research, 37(15), pp. 3704–3708. doi.org/10.1016/S0043-1354(03)00245-8.
- Van Heerden, J., et al. (2004) Prevalence of human adenoviruses in raw and treated water. Water Science & Technology, 50(1), pp. 39–43. doi.org/10.2166/wst.2004.0013.
- Villena, C., et al. (2003) A large infantile gastroenteritis outbreak in Albania caused by multiple emerging rotavirus genotypes. Epidemiology & Infection, 131(3), pp. 1105–1110. doi.org/10.1017/S0950268803001353.
- Mena, K D & Gerba, C P (2008) Waterborne Adenovirus. Reviews of Environmental Contamination and Toxicology, Springer, pp. 133–167. doi.org/10.1007/978-0-387-09647-6_4.
- Crabtree, K., et al. (1997) Waterborne adenovirus: a risk assessment. Water Science & Technology, 35(11–12), pp. 1–6. doi.org/10.1016/S0273-1223(97)00225-4.
- Gerba, C & Enriquez, C (1997) Virus-Associated Outbreaks in Swimming Pools. Water chemistry and disinfection: swimming pools and spas. National Spa and Pool Institute, Alexandria, VA, pp. 31–45.
- Divizia, M., et al. (2004) Waterborne gastroenteritis outbreak in Albania. Water Science Technology, 50(1), pp. 57–61. doi.org/10.2166/wst.2004.0018.
- Lennon, G., et al. (2007) Prevalence and characterization of enteric adenoviruses in the South of Ireland. Journal of Medical Virology, 79(10), pp. 1518–1526. doi.org/10.1002/jmv.20975.
- Li, F., et al. (2005) Structure of SARS coronavirus spike receptor-binding domain complexed with receptor. Science, 309(5742), pp. 1864–1868. doi.org/10.1126/science.1116480.
- Zhong, N S & Zeng, G Q (2003) Our strategies for fighting severe acute respiratory syndrome (SARS). American Journal of Respiratory and Critical Care Medicine, 168(1), pp. 7–9. doi.org/10.1164/rccm.200305-707OE.
- Lam, W., et al. (2003) Overview on SARS in Asia and the world. Respirology, 8, pp. S2–S5. doi.org/10.1046/j.1440-1843.2003.00516.x.
- Hui, D. S., et al. (2020) The continuing 2019-nCoV epidemic threat of novel coronaviruses to global health-the latest 2019 novel coronavirus outbreak in Wuhan China. International Journal of Infectious Diseases, 91, pp. 264–266. doi.org/10.1016/j.ijid.2020.01.009.
- Riou, J & Althaus, C L (2020) Pattern of early human-to-human transmission of Wuhan 2019 novel coronavirus (2019-nCoV), December 2019 to January 2020. Eurosurveillance, 25(4).
- Singh, L., et al. (2017) The role of nanotechnology in the treatment of viral infections. Therapeutic Advances in Infectious Disease, 4(4), pp. 105–131. doi.org/10.1177/2049936117713593.
- Matharu, R. K., et al. (2018) Nanocomposites: suitable alternatives as antimicrobial agents. Nanotechnology, 29(28), p. 282001. doi.org/10.1088/1361-6528/aabbff.
- Li, J., et al. (2020) AVNP2 protects against cognitive impairments induced by C6 glioma by suppressing tumour associated inflammation in rats. Brain Behavior and Immunity, 87, pp. 645–659. doi.org/10.1016/j.bbi.2020.02.009.
- Saxena, A., et al. (2021) Recent advances in materials science: a reinforced approach toward challenges against COVID-19. Emergent materials, 4, pp. 1–17. doi.org/10.1007/s42247-021-00179-5.
- Vahedifard, F & Chakravarthy, K (2021) Nanomedicine for COVID-19: the role of nanotechnology in the treatment and diagnosis of COVID-19. Emergent materials, 4, pp. 75–99. doi.org/10.1007/s42247-021-00168-8.
- Matharu, R. K., et al., (2020) Microstructure and antibacterial efficacy of graphene oxide nanocomposite fibres. Journal of Colloid and Interface Science, 571, pp. 239–252. doi.org/10.1016/j.jcis.2020.03.037.
- Matharu, R. K., et al., (2018) Antimicrobial activity of tellurium-loaded polymeric fiber meshes. Journal of Applied Polymer Science, 135(25), p. 46368. doi.org/10.1002/app.46368.
- Matharu, R. K., et al., (2018) The effect of graphene–poly (methyl methacrylate) fibres on microbial growth. Interface Focus, 8(3), p. 20170058. doi.org/10.1098/rsfs.2017.0058.
- Matharu, R. K., et al., (2020) Comparative study of the antimicrobial effects of tungsten nanoparticles and tungsten nanocomposite fibres on hospital acquired bacterial and viral pathogens. Nanomaterials, 10(6), p. 1017. doi.org/10.3390/nano10061017.
- Alenezi, H (2019) Experimental and theoretical investigation of the fluid behavior during polymeric fiber formation with and without pressure. Applied Physics Reviews, 6(4), p. 041401. doi.org/10.1063/1.5110965.
- Baer, A & Kehn-Hall, K (2014) Viral concentration determination through plaque assays: using traditional and novel overlay systems. Journal of Visualized Experiments, (93), p. e52065. doi.org/10.3791%2F52065.
- Smither, S. J., et al. (2013) Comparison of the plaque assay and 50% tissue culture infectious dose assay as methods for measuring filovirus infectivity. Journal of Virological Methods, 193(2), pp. 565–571. doi.org/10.1016/j.jviromet.2013.05.015.
- Max, J-J & Chapados, C (2009) Isotope effects in liquid water by infrared spectroscopy. III. H2O and D2O spectra from 6000to0cm−1. Journal of Chemical Physics, 131(18), p. 184505. doi.org/10.1063/1.3258646.
- Wang, C.-Y., et al. (2015) Influence of gas packing and orientation on FTIR activity for CO chemisorption to the Cu paddlewheel. Physical Chemistry Chemical Physics, 17(40), pp. 26766–26776. doi.org/10.1039/C5CP04474J.
- Tuschel, D (2017) Why are the Raman spectra of crystalline and amorphous solids different? Spectroscopy, 32(3), pp. 26–33.
- Durmuş, S & Ülgen, K Ö (2017) Comparative interactomics for virus-human protein-protein interactions: DNA viruses versus RNA viruses. FEBS Open Bio, 7(1), pp. 96–107. doi.org/10.1002/2211-5463.12167.
- Duffy, S (2018) Why are RNA virus mutation rates so damn high? PLOS Biology, 16(8), p. e3000003. doi.org/10.1371/journal.pbio.3000003.
- Graci, J D & Cameron, C E (2008) Therapeutically targeting RNA viruses via lethal mutagenesis. Future Virology, 3(6), pp. 553–566. doi.org/10.2217/17460794.3.6.553.
- Galdiero, S., et al. (2011) Silver nanoparticles as potential antiviral agents. Molecules, 16(10), pp. 8894–8918. doi.org/10.3390/molecules16108894.
- Baram-Pinto, D., et al. (2009) Inhibition of herpes simplex virus type 1 infection by silver nanoparticles capped with mercaptoethane sulfonate. Bioconjugate Chemistry, 20(8), pp. 1497–1502. doi.org/10.1021/bc900215b.
- Huy, T. Q., et al. (2017) Cytotoxicity and antiviral activity of electrochemical–synthesized silver nanoparticles against poliovirus. Journal of Virological Methods, 241, pp. 52–57. doi.org/10.1016/j.jviromet.2016.12.015.
- Fujimori, Y., et al. (2012) Novel antiviral characteristics of nanosized copper (I) iodide particles showing inactivation activity against 2009 pandemic H1N1 influenza virus. Applied Environmental Microbiology, 78(4), pp. 951–955. doi.org/10.1128/AEM.06284-11.
- Merkl, P., et al. (2021) Antiviral activity of silver, copper oxide and zinc oxide nanoparticle coatings against SARS-CoV-2. Nanomaterials, 11(5), p. 1312. doi.org/10.3390/nano11051312.
- Kümel, G., et al. (1990) The mechanism of the antiherpetic activity of zinc sulphate. Journal of General Virology, 71(12), pp. 2989–2997. doi.org/10.1099/0022-1317-71-12-2989.
- Suara, R O & Crowe, J E (2004) Effect of zinc salts on respiratory syncytial virus replication. Antimicrobial Agents and Chemotherapy, 48(3), pp. 783–790. doi.org/10.1128/AAC.48.3.783-790.2004.
- Tavakoli, A., et al. (2018) Polyethylene glycol-coated zinc oxide nanoparticle: an efficient nanoweapon to fight against herpes simplex virus type 1. Nanomedicine, 13(21), pp. 2675–2690. doi.org/10.2217/nnm-2018-0089.
- Park, S. E., et al. (2009) Mechanical properties of surface-charged poly(methyl methacrylate) as denture resins. International Journal of Dentistry, pp. 841431–841431. doi.org/10.1155/2009/841431.
- Karlin, S & Brendel, V (1988) Charge configurations in viral proteins. Proceedings of the National Academy of Sciences, 85(24), pp. 9396–9400. doi.org/10.1073/pnas.85.24.9396.
- Shi, H & Tarabara, V V (2018) Charge, size distribution and hydrophobicity of viruses: effect of propagation and purification methods. Journal of Virological Methods, 256, pp. 123–132. doi.org/10.1016/j.jviromet.2018.02.008.
- Carrola, J., et al. (2016) Metabolomics of silver nanoparticles toxicity in HaCaT cells: structure–activity relationships and role of ionic silver and oxidative stress. Nanotoxicology, 10(8), pp. 1105–1117. doi.org/10.1080/17435390.2016.1177744.
- Kim, M J & Shin, S (2014) Toxic effects of silver nanoparticles and nanowires on erythrocyte rheology. Food and Chemical Toxicology, 67, pp. 80–86. doi.org/10.1016/j.fct.2014.02.006.
- Sabella, S., et al. (2014) A general mechanism for intracellular toxicity of metal-containing nanoparticles. Nanoscale, 6(12), pp. 7052–7061. doi.org/10.1039/C4NR01234H.
- Lin, L., et al. (2018) Silver nanoparticles induce SH-SY5Y cell apoptosis via endoplasmic reticulum-and mitochondrial pathways that lengthen endoplasmic reticulum-mitochondria contact sites and alter inositol-3-phosphate receptor function. Toxicology Letters, 285, pp. 156–167. doi.org/10.1016/j.toxlet.2018.01.004.
- Sahu, S. C., et al. (2014) Comparative cytotoxicity of nanosilver in human liver HepG2 and colon Caco2 cells in culture. Journal of Applied Toxicology, 34(11), pp. 1155–1166. doi.org/10.1002/jat.2994.
- Shi, J., et al. (2014) Endothelial cell injury and dysfunction induced by silver nanoparticles through oxidative stress via IKK/NF-κB pathways. Biomaterials, 35(24), pp. 6657–6666. doi.org/10.1016/j.biomaterials.2014.04.093.
- Jiang, X., et al. (2018) Nanotoxicity of silver nanoparticles on HEK293T cells: a combined study using biomechanical and biological techniques. ACS Omega, 3, pp. 6770–6778. doi.org/10.1021/acsomega.8b00608.
- Vidanapathirana, A. K., et al. (2018) Acute intravenous exposure to silver nanoparticles during pregnancy induces particle size and vehicle dependent changes in vascular tissue contractility in Sprague Dawley rats. Reproductive Toxicology, 75, pp. 10–22. doi.org/10.1016/j.reprotox.2017.11.002.
- Alessandrini, F., et al. (2017) Pro-inflammatory versus immunomodulatory effects of silver nanoparticles in the lung: the critical role of dose, size and surface modification. Nanomaterials, 300. doi.org/10.3390/nano7100300.
- Trickler, W. J., et al. (2010) Silver nanoparticle induced blood-brain barrier inflammation and increased permeability in primary rat brain microvessel endothelial cells. Toxicological Sciences, 118, pp. 160–170. doi.org/10.1093/toxsci/kfq244.
- Sokołowska, P., et al. (2017) Human brain endothelial barrier cells are distinctly less vulnerable to silver nanoparticles toxicity than human blood vessel cells: a cell-specific mechanism of the brain barrier? Nanomedicine: Nanotechnology biology and medicine, 13, pp. 2127–2130. doi.org/10.1016/j.nano.2017.05.015.
- Butler, K. S., et al. (2015) Silver nanoparticles: correlating nanoparticle size and cellular uptake with genotoxicity. Mutagenesis, 30, pp. 577–591. doi.org/10.1093/mutage/gev020.
- Jeong, Y., et al. (2014) Assessment of size-dependent antimicrobial and cytotoxic properties of silver nanoparticles. Advances in Materials Science and Engineering, 2014, p. 763807. doi.org/10.1155/2014/763807.
- Kokura, S., et al. (2010) Silver nanoparticles as a safe preservative for use in cosmetics. Nanomedicine: Nanotechnology. Biology and Medicine, 6, pp. 570–574. doi.org/10.1016/j.nano.2009.12.002.
- Burdușel, A. C., et al. (2018) Biomedical applications of silver nanoparticles: an up-to-date overview. Nanomaterials, 8(9), p. 681. doi.org/10.3390/nano8090681.
- Konop, M., et al. (2016) Certain aspects of silver and silver nanoparticles in wound care: a minireview. Journal of Nanomaterials, 7614753. doi.org/10.1155/2016/7614753.
- Tian, J., et al. (2007) Topical delivery of silver nanoparticles promotes wound healing. ChemMedChem, 2, pp. 129–136. doi.org/10.1002/cmdc.200600171.
- Boca, S. C., et al. (2011) Chitosan-coated triangular silver nanoparticles as a novel class of biocompatible, highly effective photothermal transducers for in vitro cancer cell therapy. Cancer Letters, 311, pp. 131–140. doi.org/10.1016/j.canlet.2011.06.022.
- Boca-Farcau, S., et al. (2014) Folic acid-conjugated, SERS-labeled silver nanotriangles for multimodal detection and targeted photothermal treatment on human ovarian cancer cells. Molecular Pharmaceutics, 11, pp. 391–399. doi.org/10.1021/mp400300m.
- Alarcon, E. I., et al. (2016) Coloured cornea replacements with anti-infective properties: expanding the safe use of silver nanoparticles in regenerative medicine. Nanoscale, 8, pp. 6484–6489. doi.org/10.1039/C6NR01339B.
- Lu, L., et al. (2008) Silver nanoparticles inhibit hepatitis B virus replication. Antiviral Therapy, 13(2), p. 253. doi.org/10.1177/135965350801300210.
- Lara, H. H., et al. (2010) Mode of antiviral action of silver nanoparticles against HIV-1. Journal of Nanobiotechnology, 8(1), p. 1. doi.org/10.1186/1477-3155-8-1.